Late-Quaternary changes in arctic terrestrial ecosystems, climate, and ultraviolet radiation levels
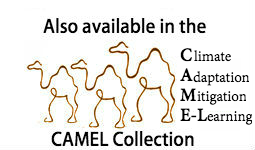
This is Section 7.2 of the Arctic Climate Impact Assessment
Lead Author: Terry V. Callaghan; Contributing Authors: Lars Olof Björn, F. Stuart Chapin III,Yuri Chernov,Torben R. Christensen, Brian Huntley, Rolf Ims, Margareta Johansson, Dyanna Jolly Riedlinger, Sven Jonasson, Nadya Matveyeva,Walter Oechel, Nicolai Panikov, Gus Shaver; Consulting Authors: Josef Elster, Heikki Henttonen, Ingibjörg S. Jónsdóttir, Kari Laine, Sibyll Schaphoff, Stephen Sitch, Erja Taulavuori, Kari Taulavuori, Christoph Zöckler
In order to understand the present biota and ecosystems of the Arctic, and to project the nature of their responses to potentially rapid future climate change, it is necessary to examine at least the last 21,000 years of their history. This period, which is part of the late Quaternary Period, extends from the present back to the last glacial maximum (LGM), encompassing the Holocene, or postglacial period, that spans approximately the last 11,400 years. A review of this period of the history of the biota and ecosystems found in the Arctic today also must examine a spatial domain that is not restricted to the present arctic regions. At the LGM, many of these regions were submerged beneath vast ice sheets, whereas many of the biota comprising present arctic ecosystems were found at lower latitudes.
Contents
Environmental history (7.2.1)
At the LGM, vast ice sheets accumulated not only on many high-latitude continental areas but also across some relatively shallow marine basins. The beds of relatively shallow seas such as the North Sea (North Sea, Europe) and Bering Sea were exposed as a result of a global sea-level fall of approximately 120 meters (m), resulting in a broad land connection between eastern Siberia and Alaska and closure of the connection between the Pacific and Arctic Oceans. The reduction in sea level also exposed a broad strip of land extending northward from the present coast of Siberia. Most, if not all, of the Arctic Ocean basin may have been covered by permanent sea ice.
Although details of the extent of some of the ice sheets continue to be a controversial matter[1], it is certain that the majority of land areas north of 60° N were ice-covered. The principal exceptions were in eastern Siberia, Beringia, and Alaska, although there is some geological evidence to suggest that smaller ice-free areas also persisted in the high Arctic, for example in the northernmost parts of the Canadian Archipelago[2] and perhaps even in northern and northeastern Greenland[3]. This evidence is supported by recent molecular genetic studies of arctic species; for example, a study of the dwarf shrub Dryas integrifolia indicates glacial occurrences in the high Arctic[4] as well as in Beringia, and a study of the collared lemming Dicrostonyx groenlandicus indicates separate glacial populations east and west of the Mackenzie River[5], the latter most probably in the Canadian Archipelago. The latter conclusion is supported by the phylogeography (relationship between genetic identity and geographic distribution) of the Paranoplocephala arctica species complex, a cestode parasite of Dicrostonyx spp., indicating that two subclades probably survived the LGM with their host in the Canadian High Arctic[6]. More controversial are suggestions that elements of the arctic flora and fauna may have survived the LGM on nunataks (hills or mountains extending above the surface of a glacier) in glaciated areas of high relief such as parts of Greenland, Svalbard, and Iceland [7]. Although a recent molecular genetic study of the alpine cushion plant Eritrichium nanum[8] provides strong evidence for survival of that species on nunataks within the heart of the European Alps, similar studies of arctic species have so far not supported the hypothesis of survival on nunataks in areas such as Svalbard[9] that experienced extreme climatic severity as ice sheets extended to margins beyond the current coast during the LGM.
Direct evidence of the severity of the full glacial climate in the Arctic comes from studies of ice cores from the Greenland Ice Sheet and other arctic ice sheets (Section 2.7 (Late-Quaternary changes in arctic terrestrial ecosystems, climate, and ultraviolet radiation levels)) that indicate full glacial conditions with mean annual temperatures 10 to 13 °C colder than during the Holocene[10]. Paleotemperature reconstructions based upon dinoflagellate cyst assemblages indicate strong seasonal temperature fluctuations, with markedly cold winter temperatures[11].
The LGM was, however, relatively short-lived; within a few millennia of reaching their maximum extent many of the ice sheets were decaying rapidly and seasonal temperatures had increased in many parts of the Arctic. Deglaciation was not, however, a simple unidirectional change; instead a series of climatic fluctuations occurred during the period between about 18,000 and 11,400 years before present (BP) that varied in intensity, duration, and perhaps also in geographic extent. The most marked and persistent of these fluctuations, the Younger Dryas event[12], was at least hemispheric in its extent, and was marked by the reglaciation of some regions and readvances of ice-sheet margins in others. Mean annual temperatures during this event fell substantially; although not as low as during the glacial maximum, they were nonetheless 4 to 6 °C cooler than at present over most of Europe[14], and as much as 10 to 12 °C colder than at present in the northern North Atlantic and the Norwegian Sea[15], as well as in much of northern Eurasia[16]. The end of the Younger Dryas was marked by a very rapid rise in temperatures. At some locations, mean annual temperature rose by more than 5 °C in less than 100 years[17]. The most rapid changes probably were spatially and temporally transgressive, with the global mean change thus occurring much less rapidly. Nonetheless, in many areas summer temperatures during the early Holocene rose to values higher than those at present. Winter conditions remained more severe than today in many higher-latitude areas, however, because the influence of the decaying ice sheets persisted into the early millennia of the Holocene.
Despite higher summer temperatures in the early to mid-Holocene in most of the Arctic, Holocene climate has not differed qualitatively from that at present. Following the general thermal maximum there has been a modest overall cooling trend throughout the second half of the Holocene. However, a series of millennial and centennial fluctuations in climate have been superimposed upon these general longer-term patterns[18]. The most marked of these occurred about 8,200 years BP and appears to have been triggered by the catastrophic discharge of freshwater into the northern North Atlantic from proglacial lakes in North America[19]. A reduction in strength, if not a partial shutdown, of the thermohaline circulation in the northern North Atlantic and Norwegian Sea was also associated with this event, as well as with the series of less severe climatic fluctuations that continued throughout the Holocene[[[20]]].
The most recent of these climatic fluctuations was that of the "Little Ice Age" (LIA), a generally cool interval spanning approximately the late 13th to early 19th centuries (Section 2.7.5 (Late-Quaternary changes in arctic terrestrial ecosystems, climate, and ultraviolet radiation levels)). At its most extreme, mean annual temperatures in some arctic areas fell by several degrees. Sea ice extended around Greenland and in some years filled the Denmark Strait between Greenland and Iceland[21], the Norse settlement of Greenland died out[22], and the population of Iceland was greatly reduced[23]. Although there was great temporal climate variability (on decadal to centennial timescales) within the LIA, and spatial variability in the magnitude of the impacts, it was apparently a period of generally more severe conditions in arctic and boreal latitudes; the marked impacts upon farming and fisheries[24] imply similar impacts on other components of the arctic ecosystem. Since the early 19th century, however, there has been an overall warming trend[25], although with clear evidence of both spatial variability and shorter-term temporal variability[26]. The magnitude of this recent warming is comparable to that of the warmest part of the Holocene, at least in those parts of the Arctic that have experienced the most rapid warming during the last 30 years or so.
The solar variability thought to be responsible for the LIA, and for other similar centennial to millennial climatic fluctuations, probably also affected the ozone layer and UV-B radiation levels. Ultraviolet-B irradiance at ground level absorbed by DNA could have been between 9 and 27% higher during periods of low solar output (cool periods) than during periods of high solar output[27] (Section 5.4.1 (Late-Quaternary changes in arctic terrestrial ecosystems, climate, and ultraviolet radiation levels)).
History of arctic biota (7.2.2)
During the LGM, when most land areas in the Arctic were ice-covered, biomes able to support the elements of the arctic biota, including some species that are now extinct, were extensive south of the Fennoscandian Ice Sheet in Europe[28]. Similar biomes apparently were extensive south of the Eurasian ice sheets of northern Russia, eastward across Siberia and the exposed seabed to the north, and via Beringia into Alaska and the northern Yukon[29], although they were much more restricted south of the Laurentide Ice Sheet in central and eastern North America[30]. The most extensive and important of these glacial biomes, the steppe–tundra, has been interpreted and referred to by various authors as "tundra–steppe" or "Mammoth steppe"[31]. The vegetation of this biome comprised a no-analogue combination of light-demanding herbaceous and dwarf-shrub taxa that are found today either in arctic tundra regions or in the steppe regions that characterize central parts of both North America and Eurasia[32]. Evidence of an abundance of grazing herbivores of large body mass, some extant (e.g., reindeer/caribou – Rangifer tarandus; muskox – Ovibos moschatus) and others extinct (e.g., giant deer or "Irish elk" – Megaloceros giganteus; woolly mammoth – Mammuthus primigenius; woolly rhinoceros – Coelodonta antiquitatis), associated with this biome suggests that it was much more productive than is the contemporary tundra biome. This productive biome, dominated by non-tree taxa, corresponded to a no-analogue environment that was relatively cold throughout the year, with a growing season short enough to exclude even cold-tolerant boreal trees from at least the majority of the landscape. The "light climate", however, was that of the relatively lower latitudes (as low as 45° N in Europe) at which this biome occurred, rather than that of the present arctic latitudes; the greater solar angle and consequent higher insolation intensities during the summer months probably made an important contribution to the productivity of the biome.
The productive steppe–tundra and related biomes were much more spatially extensive during the last glacial stage than is the tundra biome today (Fig. 7.4). The last glacial stage was thus a time when many elements of the present arctic biota thrived, almost certainly in greater numbers than today. Fossil remains of both arctic plants[33]) and mammals[34] found at numerous locations attest to their widespread distribution and abundance. Similar conclusions have been reached on the basis of phylogeographic studies of arctic-breeding waders[35]. Species such as red knot (Calidris canutus) and ruddy turnstone (Arenaria interpres) are inferred to have had much larger populations and more extensive breeding areas during glacial stages, although others, such as dunlin (C. alpina), exhibit evidence of range fragmentation during glacial stages leading to the evolution of distinct geographically restricted infraspecific taxa. Phylogeographic studies of other arctic taxa show individualistic responses (see [36] for a recent review). Some species, such as Arctic char (Salvelinus alpinus [37]), and genera, such as whitefish (Coregonus spp. [38]), exhibit evidence of sub-taxa whose origins are apparently related to recurrent isolation of populations throughout the alternating glacial and interglacial stages of the Pleistocene. Collared lemmings (Dicrostonyx spp.), however, apparently parallel C. alpina in exhibiting genetic differentiation principally as a consequence of the relatively recent geographic isolation of populations during the last glacial stage[39]. Other species, such as the polar bear (Ursus maritimus [40]), exhibit little or no evidence of genetic differentiation that might indicate past population fragmentation, and Fedorov et al.[41] inferred that Eurasian true lemmings (Lemmus spp.) experienced no effective reduction in population size during recent glacial–interglacial cycles.
In the context of their late-Quaternary history, the arctic biota at present are relatively restricted in range and population size. Although tundra areas were of even smaller extent during the early part of the Holocene than at present, as a result of greater northward extension of the treeline[42], that reduction in extent was small in magnitude compared to that experienced at the end of the last glacial stage, during which they were much more extensive than at any time since. Similarly, while extant arctic taxa at the lower taxonomic levels often exhibit considerable diversity (Species diversity) that can be related to their late-Quaternary history, the biota as a whole has suffered a recent reduction in overall diversity owing to the extinctions of many species, and some genera, that did not survive into the Holocene. Of at least 12 large herbivores and six large carnivores present in steppe–tundra areas at the LGM[43], only four and three, respectively, survive today. Of the surviving species, only two herbivores (reindeer/caribou and muskox) and two carnivores (brown bear – Ursus arctos and wolf – Canis lupus) occur today in the arctic tundra biome. Present arctic geography also imposes extreme migratory distances upon many tundra-breeding birds owing to the wide separation between their breeding and wintering areas[44], rendering many of them, in common with much of the arctic biota, extremely vulnerable to any further climatic warming[45].
Ecological history (7.2.3)
Although relatively few in overall number, paleoecological studies of the late Quaternary Period have been conducted in many parts of the Arctic (see e.g., [46]). In areas that were by then ice free, the transition to the Holocene was marked by evidence of rapid ecological response. Elsewhere, in proximity to the decaying ice sheets, there was a lag between the global changes and the ecological changes because of the regional influence of the ice sheets. Although the precise nature of the ecological changes depended upon location, the overall picture was one of widespread rapid replacement of the open, discontinuously vegetated tundra and polar desert that characterized most ice-free areas during the late-glacial period by closed tundra. This was in turn replaced by shrub tundra and subsequently by arctic woodlands or northern boreal forest in southern areas of the Arctic. In areas that were unglaciated at the LGM (e.g., Alaska), the ecological transition began earlier, coinciding with the first rapid climatic warming recorded in Greenland about 14,700 years BP[47]. In Alaska, tundra was replaced by shrub tundra during the late-glacial stage, and the first forest stands (of balsam poplar – Populus balsamifera) were already present before the transition to the Holocene[48]. South of the Arctic, the extensive areas of steppe–tundra that were present at the LGM were rapidly replaced by expanding forests. Only in parts of northernmost Siberia may fragments of the steppe–tundra biome have persisted into the Holocene, supporting the last population of woolly mammoths that persisted as recently as 4,000 years BP[49].
The early Holocene was characterized by higher summer insolation intensities at northern latitudes than at present. The warmer summer months enabled trees to extend their ranges further northward than at present; positive feedback resulting from the contrasting albedo of forest compared to tundra (Sections 7.4.2.4 and 7.5.4.2 (Late-Quaternary changes in arctic terrestrial ecosystems, climate, and ultraviolet radiation levels)) probably enhanced this extension of the forest[50]. Boreal forest trees expanded their ranges at rates of between 0.2 and 2 kilometers per year (km/yr)[51]. They exhibited individualistic responses with respect to their distributions and abundance patterns in response to climatic patterns that differed from those of today. Milder winters and more winter precipitation in western Siberia during the early Holocene, for example, allowed Norway spruce (Picea abies) to dominate in areas where Siberian fir (Abies sibirica) and Siberian stone pine (Pinus sibirica) have become important forest components during the later Holocene[52]. Throughout northern Russia, the arctic treeline had advanced more or less to the position of the present arctic coastline by about 10,200 years BP, although the lower sea level at that time meant that a narrow strip of tundra, up to 150 km wide at most, persisted north of the treeline[53]. Subsequently, as sea level continued to rise during the early Holocene, tundra extent reached a minimum that persisted for several millennia. For tundra species, including tundra-breeding birds, the early Holocene thus seems likely to have been a time of particular stress. This stress may, however, have been in part relieved by enhanced productivity in these areas, compared to modern tundra ecosystems, as a consequence of the warmer summers and higher insolation intensity.
In glaciated areas of the Arctic, such as northern Fennoscandia and much of arctic Canada, peatlands became extensive only after the mid-Holocene (see e.g., [54]) in response to the general pattern of climatic change toward cooler and regionally moister summer conditions. The same cooling trend led to the southward retreat of the arctic treeline, which reached more or less its present location in most regions by about 4,500 years BP[55]. The consequent increase in tundra extent probably relieved the stress experienced by tundra organisms during the early Holocene, although the cooler, less productive conditions, and the increasing extent of seasonally waterlogged tundra peatlands, may have offset this at least in part. While the early Holocene was a time of permafrost decay and thermokarst development, at least in some regions[56], the extent of permafrost has increased in many areas during the later Holocene (see e.g., [57]).
Recently discovered evidence[58] shows that Paleolithic "hunter-gatherers" were present about 40,000 years BP (long before the LGM) as far north as 66°34' N in Russia, east of the Fennoscandian Ice Sheet. Although it seems likely that humans did not range as far north during the glacial maximum, it is clear that they expanded rapidly into the Arctic during the deglaciation.
Humans entered North America via the Bering "land bridge" and along the southern coast of Beringia about 14,000 to 13,500 years BP[59]. These so-called Clovis hunters were hunter-gatherers who had developed sophisticated ways of working stone to produce very fine spear- and arrowheads. Over the next few millennia, they expanded their range and population rapidly, occupying most of the North American continent. Their prey apparently included many of the large vertebrate species that soon became extinct. The extent to which human hunting may have been principally responsible for these extinctions is a matter of continuing debate, but recent simulations for North America indicate that this possibility cannot be excluded[60]. However, these extinctions also coincide with an environmental change that caused the area of the biome with which the large arctic vertebrates were associated to be reduced to an extent that was apparently unprecedented during previous glacial–interglacial cycles[61]. It thus is more probable that the hunting pressure exerted by humans was at most an additional contributory factor leading to the extinctions, rather than their primary cause.
In Eurasia, Paleolithic hunter-gatherers shifted their range northward into the Arctic at the end of the last glacial stage, as did their large vertebrate prey. To the south, they were replaced by Mesolithic peoples who occupied the expanding forests. By the early Holocene these Mesolithic peoples had expanded well into the Arctic[62], where they probably gave rise to the indigenous peoples that in many cases continued to practice a nomadic hunter-gatherer way of life until the recent past or even up to the present day in some regions. The arrival of later immigrants has had major impacts upon indigenous peoples and their way of life (Chapters 3, 11, and 12 (Late-Quaternary changes in arctic terrestrial ecosystems, climate, and ultraviolet radiation levels)). In turn, land use and natural resource exploitation by the immigrants, as well as the changes that they have brought about in the way of life of indigenous peoples, have had negative impacts on many arctic ecosystems. These impacts in some cases have possibly increased the vulnerability of these ecosystems to the pressures that they now face from climate change and increased exposure to UV-B radiation.
Future change in the context of late-Quaternary changes (7.2.5)
The potential changes for the next century can be put into context by comparing their rates and magnitudes to those estimated for the changes documented by paleoecological and other evidence from the late Quaternary Period (Table 7.1).
Late Quaternary |
Projected Future | |
Sea Level |
ca. 120 m lower at LGM; increased at a maximum rate of ca. 24 mm/yr [63] |
0.09–0.88 m higher by 2100; 3–10 m higher in 1000 years increasing at a rate of 1-9 mm/yr [64] |
Climate |
||
Mean annual temperature |
full glacial: global mean ca. 5 °C lower; regionally in the Arctic 10–13 °C lower Holocene: global mean <1 °C higher at maximum; regionally in the Arctic similar to present |
2100: global mean 1.5–5.8 ºC higher; regionally in the Arctic 2.1&ndash8.1 °C higher [65] |
Winter temperature |
full glacial: >15 °C cooler regionally in the Arctic Holocene: ca. 2–4 &ndegC warmer regionally in the Arctic at maximum |
2100: 4–10 °C higher regionally in the Arctic [66] |
Rate of increase in mean annual temperature |
global: ?1 °C per millennium; regionally in the Arctic: >5 °C in a century |
global: 1.5–5.8 oC per century; regionally in the Arctic: 2.1–8.1 °C in a century [67] |
Ecosystem responses |
||
Treeline displacement |
full glacial: >1000 km southward; Holocene: 50–200 km northward at maximum [68] |
2100: >500 km northward. It is possible that anthropogenic disturbance might result in an opposite response (see Section 7.5.3.2 (Late-Quaternary changes in arctic terrestrial ecosystems, climate, and ultraviolet radiation levels)) |
Range margin displacement rates |
early Holocene: rates of 0.2–2 km/yr estimated for trees from pollen data [69] |
21st century: potential rates of 5–10 km/yr estimated from species–climate response models [70] |
Area of tundra |
full glacial: 197% (ranging from 168 to 237%) of present; Holocene: 81% (ranging from 76 to 84%) of present at minimum |
2100: 51% of present [71] |
UV-B radiation levels |
No long-term trend known. Due to solar variability, levels of DNA-active UV-B wavelengths may have varied by up to 27% within a period of ca. 150 years [72] |
In addition to natural solar cycles, it is very likely that anthropogenic cooling of the stratosphere will delay recovery of the ozone layer |
It is apparent from Table 7.1 that projected future changes have several characteristics that pose a particular threat to the biota and ecosystems of the Arctic. First, [[climatic change]s] over the next century are likely to be comparable in magnitude to the changes that occurred between full glacial conditions and present conditions, and greater than the maximum changes that occurred during the Holocene. Second, the global increase in mean annual temperature is projected to occur at rates that are higher than the rate of global temperature increase during the last deglaciation; in parts of the Arctic the rate of warming is likely to match the most rapid regional warming of the late Quaternary Period. Third, as a consequence of this temperature increase, and the accompanying rise in sea level, tundra extent is likely to be less than at any time during the late Quaternary Period. Fourth, global mean temperatures and mean annual temperatures in the Arctic are very likely to reach levels unprecedented in the late Quaternary Period; this is very likely to result in a rapid reduction in the extent of permafrost, with associated thermokarst development in areas of permafrost decay leading to potentially severe erosion and degradation of many arctic peatlands (Section 7.5.3.1 (Late-Quaternary changes in arctic terrestrial ecosystems, climate, and ultraviolet radiation levels)). The combination of projected future climate change with other anthropogenic effects (including enhanced levels of UV-B radiation, deposition of nitrogen compounds from the atmosphere, heavy metal and acidic pollution, radioactive contamination, and increased habitat fragmentation suggests that the future is very likely to be without a past analogue and will pose unprecedented challenges to arctic ecosystems and biota that evolved in response to global cooling throughout the last five million years or so (the late Tertiary and Quaternary Periods), during which our own species also evolved.
Summary (7.2.6)
At the LGM, vast ice sheets covered many continental areas. The beds of some shallow seas were exposed, connecting previously separated landmasses. Although some areas were ice-free and supported a flora and fauna, mean annual temperatures were 10 to 13 °C colder than during the Holocene. Within a few millennia of the glacial maximum, deglaciation started but was not a simple unidirectional change: a series of climatic fluctuations occurred between about 18,000 and 11,400 years BP. During the Younger Dryas event, mean annual temperatures fell substantially in some areas and reglaciation occurred. At the end of the event, mean annual temperatures rose by more than 5 °C in less than 100 years in at least some parts of the Arctic. Following the general thermal maximum in the Holocene, there has been a modest overall cooling trend. However, superimposed upon the general longer-term patterns have been a series of millennial and centennial fluctuations in climate, the most marked of which occurred about 8,200 years BP. The most recent of these climatic fluctuations was that of the LIA, a generally cool interval spanning approximately the late 13th to early 19th centuries. At its most extreme, mean annual temperatures in some arctic areas fell by several degrees, with impacts on human settlements in the north.
In the context of at least the last 150,000 years, arctic ecosystems and biota have been close to their minimum extent within the last 10,000 years. They suffered loss of diversity as a result of extinctions during the rapid, large-magnitude global warming at the end of the last glacial stage. Consequently, arctic ecosystems and biota are already stressed; some are extremely vulnerable to current and projected future climate change. For example, migratory arctic-breeding birds today face maximal migration distances between their wintering and breeding areas. Evidence from the past indicates that arctic species, especially larger vertebrates, are very likely to be vulnerable to extinction if climate warms. The treeline is very likely to advance, perhaps rapidly, into tundra areas of northern Eurasia, Canada, and Alaska, as it did during the early Holocene, reducing the extent of tundra and contributing to the pressure upon species that makes their extinction possible. Species that today have more southerly distributions are very likely to extend their ranges north, displacing arctic species. Permafrost is very likely to decay and thermokarst develop, leading to erosion and degradation of arctic peatlands. Unlike the early Holocene, when lower relative sea level allowed a belt of tundra to persist around at least some parts of the Arctic Basin when treelines advanced to the present coast, sea level is very likely to rise in the future, further restricting the area of tundra and other treeless arctic ecosystems. The negative response of arctic ecosystems in the face of a shift to global climatic conditions that are apparently without precedent during the Pleistocene is likely to be considerable, particularly as their exposure to cooccurring environmental changes (i.e., enhanced levels of UV-B radiation, deposition of nitrogen compounds from the atmosphere, heavy metal and acidic pollution, radioactive contamination, increased habitat fragmentation) is also without precedent.
Chapter 7: Arctic Tundra and Polar Desert Ecosystems
7.1 Introduction (Late-Quaternary changes in arctic terrestrial ecosystems, climate, and ultraviolet radiation levels)
7.2 Late-Quaternary changes in arctic terrestrial ecosystems, climate, and ultraviolet radiation levels
7.3 Species responses to changes in climate and ultraviolet-B radiation in the Arctic
7.3.1 Implications of current species distributions for future biotic change
7.3.2 General characteristics of arctic species and their adaptations in the context of changes in climate and ultraviolet-B radiation levels
7.3.3 Phenotypic responses of arctic species to changes in climate and ultraviolet-B radiation
7.3.4 Genetic responses of arctic species to changes in climate and ultraviolet-B radiation levels
7.3.5 Recent and projected changes in arctic species distributions and potential ranges
7.4 Effects of changes in climate and UV radiation levels on structure and function of arctic ecosystems in the short and long term
7.4.1 Ecosystem structure
7.4.2 Ecosystem function
7.5 Effects of climate change on landscape and regional processes and feedbacks to the climate system
7.6 Synthesis: Scenarios of projected changes in the four ACIA regions for 2020, 2050, and 2080
7.7 Uncertainties and recommendations
References
- #^ Walker, M.J.C., 1995. Climatic changes in Europe during the last glacial/interglacial transition. Quaternary International, 28:63–76.
- #^ Koç, N., E. Jansen, M. Hald and L. Labeyrie, 1996. Late glacial–Holocene sea surface temperatures and gradients between the North Atlantic and the Norwegian Sea: implications for the nordic heat pump. In: J.T. Andrews, W.E.N. Austin, H.E. Bergsten and A.E. Jennings (eds.). Late Quaternary Palaeoceanography of the North Atlantic Margins. Geological Society Special Publication 111:177–185.
- #^ Velichko, A.A., 1995. The Pleistocene termination in Northern Eurasia. Quaternary International, 28:105–111.
- #^ Dansgaard,W., J.W.C.White and S.J. Johnsen, 1989. The abrupt termination of the Younger Dryas climate event. Nature, 339:532–534.
- #^ Huntley, B., M. Baillie, J.M. Grove, C.U. Hammer, S.P. Harrison, S. Jacomet, E. Jansen,W. Karlen, N. Koc, J. Luterbacher, J. Negendank and J. Schibler, 2002. Holocene paleoenvironmental changes in north-west Europe: Climatic implications and the human dimension. In: G.Wefer, W.H. Berger, K.-E. Behre, and E. Jansen (eds.). Climate Development and History of the North Atlantic Realm, pp. 259–298. Springer-Verlag.
- #^ Barber, D.C., A. Dyke, C. Hillaire-Marcel, A.E. Jennings, J.T. Andrews, M.W. Kerwin, G. Bilodeau, R. McNeely, J. Southon, M.D. Morehead and J.-M. Gagnon, 1999. Forcing of the cold event of 8,200 years ago by catastrophic drainage of Laurentide lakes. Nature, 400:344–348.– Renssen, H., H. Goosse, T. Fichefet and J.-M. Campin, 2001. The 8.2 kyr BP event simulated by a global atmosphere-sea-ice-ocean model. Geophysical Research Letters, 28(8):1567–1570.
- #^ Bianchi, G.G. and I.N. McCave, 1999. Holocene periodicity in North Atlantic climate and deep-ocean flow south of Iceland. Nature, 397:515–517.
- #^ Lamb, H.H., 1982. Climate, History and the Modern World. Methuen, 387pp.– Ogilvie, A.E.J., 1984. The past climate and sea-ice record from Iceland. Part 1: Data to A.D. 1780. Climatic Change, 6:131–152.– Ogilvie, A.E.J. and I. Jonsdottir, 2000. Sea ice, climate, and Icelandic fisheries in the eighteenth and nineteenth centuries. Arctic, 53:383–394.– Ogilvie, A.E.J. and T. Jonsson, 2001. 'Little Ice Age' research: A perspective from Iceland. Climatic Change, 48:9–52.
- #^ Barlow, L.K., J.P. Sadler, A.E.J. Ogilvie, P.C. Buckland, T. Amorosi, J.H. Ingimundarson, P. Skidmore, A.J. Dugmore and T.H. McGovern, 1997. Interdisciplinary investigations of the end of the Norse Western Settlement in Greenland. The Holocene, 7(4):489–499.– Buckland, P.C., T. Amorosi, L.K. Barlow, A.J. Dugmore, P.A. Mayewski,T.H. McGovern, A.E.J. Ogilvie, J.P. Sadler and P. Skidmore, 1996. Bioarchaeological and climatological evidence for the fate of Norse farmers in medieval Greenland. Antiquity, 70(267):88–96.
- #^ Ogilvie, A.E.J., 1991. Climatic changes in Iceland A.D. c.865 to 1598. In: G.F. Bigelow (ed.).The Norse of the North Atlantic. Acta Archaelogica, 61:233–251.– Sveinbjarnardóttir, G., 1992. Farm Abandonment in Medieval and Post-Medieval Iceland: An Interdisciplinary Study. Oxbow Monograph 17. Oxbow Books, 192pp.
- #^ Lamb, H.H., 1982. Climate, History and the Modern World. Methuen, 387pp.
- #^ Overpeck, J., K. Hughen, D. Hardy, R. Bradley, R. Case, M. Douglas, B. Finney, K. Gajewski, G. Jacoby, A. Jennings, S. Lamoureux, A. Lasca, G. MacDonald, J. Moore, M. Retelle, S. Smith, A. Wolfe and G. Zielinski, 1997. Arctic environmental change of the last four centuries. Science, 278:1251–1256.
- #^ Maxwell, B., 1997. Recent climate patterns in the Arctic. In: W.C. Oechel,T. Callaghan, T. Gilmanov, J.I. Holten, B. Maxwell, U. Molau and B. Sveinbjornsson (eds.). Global Change and Arctic Terrestrial Ecosystems, pp. 21–46. Springer-Verlag.
- #^ Rozema, J., B. van Geel, L.O. Björn, J. Lean and S. Madronich, 2002. Toward solving the UV puzzle. Science, 296:1621–1622.
- #^ Huntley, B., M.J. Alfano, J.R.M. Allen, D. Pollard, P.C. Tzedakis, J.-L. de Beaulieu, E. Gruger and B. Watts, 2003. European vegetation during Marine Oxygen Isotope Stage-3. Quaternary Research, 59(2):195–212.
- #^ Ritchie, J.C., 1987. Postglacial Vegetation of Canada. Cambridge University Press, 178pp.
- #^ Lister, A. and P. Bahn, 1995. Mammoths. Boxtree, London, 168pp.
- #^ Guthrie, R.D., 2001. Origin and causes of the mammoth steppe: a story of cloud cover, woolly mammal tooth pits, buckles, and inside-out Beringia. Quaternary Science Reviews, 20:549–574.– Walker, D.A., J.G. Bockheim, F.S. Chapin III,W. Eugster, F.E. Nelson and C.L. Ping, 2001. Calcium-rich tundra, wildlife, and the 'Mammoth Steppe'. Quaternary Science Reviews, 20:149–163.– Yurtsev, B.A., 2001. The Pleistocene 'Tundra-Steppe' and the productivity paradox: the landscape approach. Quaternary Science Reviews, 20:165–174.
- #^ Yurtsev, B.A., 2001. The Pleistocene 'Tundra-Steppe' and the productivity paradox: the landscape approach. Quaternary Science Reviews,
- #^ West, R.G., 2000. Plant Life of the Quaternary Cold Stages: Evidence from the British Isles. Cambridge University Press, 332pp.
- #^ FAUNMAP Working Group, 1996. Spatial response of mammals to late Quaternary environmental fluctuations. Science, 272:1601–1606.– Lundelius, E.L. Jr., R.W. Graham, E. Anderson, J. Guilday, J.A. Holman, D.W. Steadman, and D.S.Webb, 1983.Terrestrial vertebrate faunas. In: S.C. Porter (ed.). Late Quaternary Environments of the United States. Vol 1: The Late Pleistocene, pp. 311–353. University of Minnesota Press.– Stuart, A.J., 1982. Pleistocene Vertebrates in the British Isles. Longman, 212pp.
- #^ Kraaijeveld, K. and E.N. Nieboer, 2000. Late Quaternary paleogeography and evolution of Arctic breeding waders. Ardea, 88(2):193–205.
- #^ Weider, L.J. and A. Hobaek, 2000. Phylogeography and Arctic biodiversity: a review. Annales Zoologici Fennici, 37(4):217–231.
- #^ Brunner, P.C., M.R. Douglas, A. Osinov, C.C. Wilson and L. Bernatchez, 2001. Holarctic phylogeography of Arctic char (Salvelinus alpinus L.) inferred from mitochondrial DNA sequences. Evolution, 55(3):573–586.
- #^ Bernatchez, L., A. Chouinard and G. Lu, 1999. Integrating molecular genetics and ecology in studies of adaptive radiation: whitefish, Coregonus sp., as a case study. Biological Journal of the Linnean Society, 68(1–2):173–194.
- #^ Fedorov, V.B. and A.V. Goropashnaya, 1999. The importance of ice ages in diversification of Arctic collared lemmings (Dicrostonyx): evidence from the mitochondrial cytochrome b region. Hereditas, 130(3):301–307.– Fedorov,V., A. Goropashnaya, G.H. Jarrell and K. Fredga, 1999b. Phylogeographic structure and mitochondrial DNA variation in true lemmings (Lemmus) from the Eurasian Arctic. Biological Journal of the Linnean Society, 66(3):357–371.
- #^ Paetkau, D., S.C. Amstrup, E.W. Born, W. Calvert, A.E. Derocher, G.W. Garner, F. Messier, I. Stirling, M.K. Taylor, Ø. Wiig and C. Strobeck, 1999. Genetic structure of the world's polar bear populations. Molecular Ecology, 8(10):1571–1584.
- #^ Fedorov, V.B., K. Fredga and G.H. Jarrell, 1999a. Mitochondrial DNA variation and the evolutionary history of chromosome races of collared lemmings (Dicrostonyx) in the Eurasian Arctic. Journal of Evolutionary Biology, 12(1):134–145.
- #^ Huntley, B., 1997. The responses of vegetation to past and future climate changes. In: W.C. Oechel, T. Callaghan, T. Gilmanov, J.I. Holten, B. Maxwell, U. Molau and B. Sveinbjornsson (eds.). Global Change and Arctic Terrestrial Ecosystems, pp. 290–311. Springer-Verlag.– Huntley, B. and R. Bradshaw, 1999. Palaeoecological evidence and opportunities in determining environmental change and ecological responses. In: M. Turunen, J. Hukkinen, O.W. Heal, J.I. Holten and N.R. Saelthun (eds.). A Terrestrial Transect for Scandinavia/Northern Europe: Proceedings of the International SCANTRAN Conference. Ecosystems Research Report 31:153–157. European Commission, Brussels.– MacDonald, G.M., A.A. Velichko, C.V. Kremenetski, O.K. Borisova, A.A. Goleva, A.A. Andreev, L.C. Cwynar, R.T. Riding, S.L. Forman, T.W.D. Edwards, R. Aravena, D. Hammarlund, J.M. Szeicz and V.N. Gattaulin, 2000. Holocene treeline history and climate change across northern Eurasia. Quaternary Research, 53(3):302–311.
- #^ Lister, A. and P. Bahn, 1995. Mammoths. Boxtree, London, 168pp.– Stuart, A.J., 1982. Pleistocene Vertebrates in the British Isles. Longman, 212pp.
- #^ Davidson, N.C., K.B. Strann, N.J. Crockford, P.R. Evans, J. Richardson, L.J. Standen, D.J. Townshend, J.D. Uttley, J.R. Wilson and A.G. Wood, 1986. The origins of Knots Calidris canutus in arctic Norway in Spring. Ornis Scandinavica, 17(2):175–179.– Wennerberg, L., 2001. Breeding origin and migration pattern of dunlin (Calidris alpina) revealed by mitochondrial DNA analysis. Molecular Ecology, 10(5):1111–1120.
- #^ Evans, P.R., 1997. Migratory birds and climate change. In: B. Huntley, W. Cramer, A.V. Morgan, H.C. Prentice and J.R.M. Allen (eds.). Past and Future Rapid Environmental Changes: The Spatial and Evolutionary Responses of Terrestrial Biota, pp. 227–238. Springer Verlag.
- #^ Bigelow, N.H., L.B. Brubaker, M.E. Edwards, S.P. Harrison, I.C. Prentice, P.M. Anderson, A.A. Andreev, P.J. Bartlein, T.R. Christensen, W. Cramer, J.O. Kaplan, A.V. Lozhkin, N.V. Matveyeva, D.F. Murray, A.D. McGuire, V.Y. Razzhivin, J.C. Ritchie, B. Smith, D.A. Walker, K. Gajewski,V. Wolf, B.H. Holmqvist, Y. Igarashi, K. Kremenetskii, A. Paus, M.F.J. Pisaric and V.S. Volkova, 2003. Climate change and Arctic ecosystems: 1. Vegetation changes north of 55°N between the last glacial maximum, mid-Holocene, and present. Journal of Geophysical Research, 108(D19):8170, doi:10.1029/2002JD002558.– Kaplan, J.O., N.H. Bigelow, I.C. Prentice, S.P. Harrison, P.J. Bartlein, T.R. Christensen, W. Cramer, N.V. Matveyeva, A.D. McGuire, D.F. Murray, V.Y. Razzhivin, B. Smith, D.A. Walker, P.M. Anderson, A.A. Andreev, L.B. Brubaker, M.E. Edwards and A.V. Lozhkin, 2003. Climate change and Arctic ecosystems: 2. Modeling, paleodata-model comparisons, and future projections. Journal of Geophysical Research, 108(D19):8171, doi:10.1029/2002JD002559.
- #^ Anderson, P.M. and L.B. Brubaker, 1993. Holocene vegetation and climate histories of Alaska. In: H.E. Wright Jr., J.E. Kutzbach, T. Webb III, W.F. Ruddiman, F.A. Street-Perrott and P.J. Bartlein (eds.). Global Climates since the Last Glacial Maximum, pp. 386–400. University of Minnesota Press.– Anderson, P.M. and L.B. Brubaker, 1994. Vegetation history of north central Alaska: A mapped summary of late-Quaternary pollen data. Quaternary Science Reviews, 13:71–92.– Lamb, H.F. and M.E. Edwards, 1988. Glacial and Holocene vegetation history: the Arctic. In: B. Huntley and T.Webb III (eds.).Vegetation History. Handbook of Vegetation Science, 7:519–555.– MacDonald, G.M., A.A. Velichko, C.V. Kremenetski, O.K. Borisova, A.A. Goleva, A.A. Andreev, L.C. Cwynar, R.T. Riding, S.L. Forman, T.W.D. Edwards, R. Aravena, D. Hammarlund, J.M. Szeicz and V.N. Gattaulin, 2000. Holocene treeline history and climate change across northern Eurasia. Quaternary Research, 53(3):302–311.– Ritchie, J.C., 1987. Postglacial Vegetation of Canada. Cambridge University Press, 178pp.
- #^ Björck, S., M.J.C. Walker, L.C. Cwynar, S. Johnsen, K.-L. Knudsen, J.J. Lowe and B. Wohlfarth, 1998. An event stratigraphy for the Last Termination in the North Atlantic region based on the Greenland ice-core record: a proposal by the INTIMATE group. Journal of Quaternary Science, 13(4):283–292.– Stuiver, M., P.M. Grootes and T.F. Braziunas, 1995. The GISP2 ‰18O climate record of the past 16,500 years and the role of the sun, ocean, and volcanoes. Quaternary Research, 44:341–354.
- #^ Anderson, P.M. and L.B. Brubaker, 1994. Vegetation history of north central Alaska: A mapped summary of late-Quaternary pollen data. Quaternary Science Reviews, 13:71–92.
- #^ Vartanyan, S.L., V.E. Garutt and A.V. Sher, 1993. Holocene dwarf mammoths from Wrangel Island in the Siberian Arctic. Nature, 362:337–340.
- #^ Foley, J.A., J.E. Kutzbach, M.T. Coe and S. Levis, 1994. Feedbacks between climate and boreal forests during the Holocene epoch. Nature, 371:52–54.
- #^ Huntley, B. and H.J.B. Birks, 1983. An Atlas of Past and Present Pollen Maps for Europe: 0–13000 Years Ago. Cambridge University Press, 667pp.– Ritchie, J.C. and G.M. MacDonald, 1986. The patterns of post-glacial spread of white spruce. Journal of Biogeography, 13:527–540.
- #^ Huntley, B., 1988. Glacial and Holocene vegetation history: Europe. In: B. Huntley and T.Webb III (ed.). Vegetation History. Handbook of Vegetation Science, 7:341–383. Kluwer Academic Publishers.– Huntley, B., 1997. The responses of vegetation to past and future climate changes. In: W.C. Oechel, T. Callaghan, T. Gilmanov, J.I. Holten, B. Maxwell, U. Molau and B. Sveinbjornsson (eds.). Global Change and Arctic Terrestrial Ecosystems, pp. 290–311. Springer-Verlag.– Huntley, B. and H.J.B. Birks, 1983. An Atlas of Past and Present Pollen Maps for Europe: 0–13000 Years Ago. Cambridge University Press, 667pp.
- #^ MacDonald, G.M., A.A. Velichko, C.V. Kremenetski, O.K. Borisova, A.A. Goleva, A.A. Andreev, L.C. Cwynar, R.T. Riding, S.L. Forman, T.W.D. Edwards, R. Aravena, D. Hammarlund, J.M. Szeicz and V.N. Gattaulin, 2000. Holocene treeline history and climate change across northern Eurasia. Quaternary Research, 53(3):302–311.
- #^ Lamb, H.F., 1980. Late Quaternary vegetational history of southeastern Labrador. Arctic and Alpine Research, 12:117–135.– Vardy, S.R., B.G. Warner and R. Aravena, 1997. Holocene climate effects on the development of a peatland on the Tuktoyaktuk Peninsula, Northwest Territories. Quaternary Research, 47(1):90–104.
- #^ MacDonald, G.M., A.A. Velichko, C.V. Kremenetski, O.K. Borisova, A.A. Goleva, A.A. Andreev, L.C. Cwynar, R.T. Riding, S.L. Forman, T.W.D. Edwards, R. Aravena, D. Hammarlund, J.M. Szeicz and V.N. Gattaulin, 2000. Holocene treeline history and climate change across northern Eurasia. Quaternary Research, 53(3):302–311.
- #^ Burn, C.R., 1997. Cryostratigraphy, paleogeography, and climate change during the early Holocene warm interval, western Arctic coast, Canada. Canadian Journal of Earth Sciences, 34:912–925.
- #^ Kienel, U., C. Siegert and J. Hahne, 1999. Late Quaternary palaeoenvironmental reconstructions from a permafrost sequence (North Siberian Lowland, SE Taymyr Peninsula) - a multidisciplinary case study. Boreas, 28(1):181–193.– Vardy, S.R., B.G. Warner and R. Aravena, 1997. Holocene climate effects on the development of a peatland on the Tuktoyaktuk Peninsula, Northwest Territories. Quaternary Research, 47(1):90–104.
- #^ Pavlov, P., J.I. Svendsen and S. Indrelid, 2001. Human presence in the European Arctic nearly 40,000 years ago. Nature, 413:64–67.
- #^ Dixon, E.J., 2001. Human colonization of the Americas: timing, technology and process. Quaternary Science Reviews, 20:277–299.
- #^ Alroy, J., 2001. A multispecies overkill simulation of the end-Pleistocene megafaunal mass extinction. Science, 292:1893–1896.
- #^ Sher, A., 1997. Late-Quaternary extinction of large mammals in northern Eurasia: A new look at the Siberian contribution. In: B. Huntley, W. Cramer, A.V. Morgan, H.C. Prentice and J.R.M. Allen (eds.). Past and Future Rapid Environmental Changes: The Spatial and Evolutionary Responses of Terrestrial Biota, pp. 319–339. Springer-Verlag.
- #^ Thommessen, T., 1996. The early settlement of northern Norway. In: L. Larsson (ed.). The Earliest Settlement of Scandinavia and its Relationship with Neighbouring Areas. Acta Archaeologica Lundensia, Series in 8°, 24:235–240. Almquist and Wiksell International.
- #^ Fairbanks, R.G., 1989. A 17,000-year glacio-eustatic sea level record: Influence of glacial melting rates on the Younger Dryas event and deep-ocean circulation. Nature, 342:637–642.
- #^ IPCC, 2001. Climate Change 2001: The Scientific Basis. Contribution of Working Group I to the Third Assessment Report of the Intergovernmental Panel on Climate Change. J.T. Houghton, Y. Ding, D.J. Griggs, M. Noguer, P.J. van der Linden, X. Dai, K. Maskell and C.A. Johnson (eds.). Cambridge University Press, 881pp.
- #^ Ibid.
- #^ Ibid.
- #^ Ibid.
- #^ Kaplan, J.O., 2001. Geophysical applications of vegetation modelling. Ph.D. Thesis, Lund University.
- #^ Huntley, B., 1988. Glacial and Holocene vegetation history: Europe. In: B. Huntley and T. Webb III (ed.). Vegetation History. Handbook of Vegetation Science, 7:341–383. Kluwer Academic Publishers.
- #^ Huntley, B., P.M. Berry, W. Cramer and A.P. McDonald, 1995. Modelling present and potential future ranges of some European higher plants using climate response surfaces. Journal of Biogeography, 22:967–1001.
- #^ Kaplan, J., 2002. pers. comm. Canadian Centre for Climate Modelling and Analysis.– Kaplan, J.O., N.H. Bigelow, I.C. Prentice, S.P. Harrison, P.J. Bartlein, T.R. Christensen, W. Cramer, N.V. Matveyeva, A.D. McGuire, D.F. Murray, V.Y. Razzhivin, B. Smith, D.A. Walker, P.M. Anderson, A.A. Andreev, L.B. Brubaker, M.E. Edwards and A.V. Lozhkin, 2003. Climate change and Arctic ecosystems: 2. Modeling, paleodata-model comparisons, and future projections. Journal of Geophysical Research, 108(D19):8171, doi:10.1029/2002JD002559.
- #^ Rozema, J., B. van Geel, L.O. Björn, J. Lean and S. Madronich, 2002. Toward solving the UV puzzle. Science, 296:1621–1622.
Citation
Committee, I. (2012). Late-Quaternary changes in arctic terrestrial ecosystems, climate, and ultraviolet radiation levels. Retrieved from http://editors.eol.org/eoearth/wiki/Late-Quaternary_changes_in_arctic_terrestrial_ecosystems,_climate,_and_ultraviolet_radiation_levels- ↑ Astakhov,V., 1998. The last ice sheet of the Kara Sea: Terrestrial constraints on its age. Quaternary International, 45/46:19–28.– Grosswald, M.G., 1988. An Antarctic-style ice sheet in the Northern Hemisphere: Toward a new global glacial theory. Polar Geography and Geology, 12:239–267.– Grosswald, M.G., 1998. Late-Weichselian ice sheets in Arctic and Pacific Siberia. Quaternary International, 45/46:3–18.– Lambeck, K., 1995. Constraints on the Late Weichselian ice sheet over the Barents Sea from observations of raised shorelines. Quaternary Science Reviews, 14:1–16.– Siegert, M.J., J.A. Dowdeswell and M. Melles, 1999. Late Weichselian glaciation of the Russian High Arctic. Quaternary Research, 52(3):273–285.- Callaghan,T.V., B.R.Werkman and R.M.M. Crawford, 2002b. The tundra-taiga interface and its dynamics: concepts and applications. Ambio Special Report, 12:6–14.
- ↑ Andrews, J.T., 1987. The late Wisconsin glaciation and deglaciation of the Laurentide ice sheet. In: W.F. Ruddiman and H.E. Wright Jr. (eds.). North America and Adjacent Oceans during the Last Deglaciation, pp. 13–37. The Geological Society of America, Boulder, Colorado.
- ↑ Funder, S., C. Hjort, J.Y. Landvik, S.-I. Nam, N. Reeh and R. Stein, 1998. History of a stable ice margin - East Greenland during the Middle and Upper Pleistocene. Quaternary Science Reviews, 17(1–3):77–123.
- ↑ Tremblay, N.O. and D.J. Schoen, 1999. Molecular phylogeography of ''Dryas integrifolia'': glacial refugia and postglacial recolonization. Molecular Ecology, 8(7):1187–1198.
- ↑ Ehrich, D.,V.B. Fedorov, N.C. Stenseth, C.J. Krebs and A. Kenney, 2000. Phylogeography and mitochondrial DNA (mtDNA) diversity in North American collared lemmings (''Dicrostonyx groenlandicus''). Molecular Ecology, 9(3):329–337.– Fedorov,V.B. and A.V. Goropashnaya, 1999. The importance of ice ages in diversification of Arctic collared lemmings (''Dicrostonyx''): evidence from the mitochondrial cytochrome b region. Hereditas, 130(3):301–307.
- ↑ Wickström, L.M.,V. Haukisalmi, S.Varis, J. Hantula,V.B. Fedorov and H. Henttonen, 2003. Phylogeography of the circumpolar ''Paranoplocephala arctica'' species complex (Cestoda: Anoplocephalidae) parasitizing collared lemmings (''Dicrostonyx'' spp.). Molecular Ecology, 12:3359–3371.
- ↑ Rundgren, M. and O. Ingolfsson, 1999. Plant survival in Iceland during periods of glaciation? Journal of Biogeography, 26(2):387–396.
- ↑ Stehlik, I., J.J. Schneller and K. Bachmann, 2001. Resistance or emigration: response of the high-alpine plant ''Eritrichium nanum'' (L.) Gaudin to the ice age within the Central Alps. Molecular Ecology, 10(2):357–370.
- ↑ Abbott, R.J., L.C. Smith, R.I. Milne, R.M.M. Crawford, K.Wolff and J. Balfour, 2000. Molecular analysis of plant migration and refugia in the Arctic. Science, 289:1343–1346.
- ↑ Grootes, P.M., M. Stuiver, J.W.C. White, S. Johnsen and J. Jouzel, 1993. Comparison of oxygen isotope records from the GISP2 and GRIP Greenland ice cores. Nature, 366:552–554.
- ↑ de Vernal, A. and C. Hillaire-Marcel, 2000. Sea-ice cover, sea-surface salinity and halo/thermocline structure of the northwest North Atlantic: modern versus full glacial conditions. Quaternary Science Reviews, 19(1–5):65–85.– de Vernal, A., C. Hillaire-Marcel, J.-L. Turon and J. Matthiessen, 2000. Reconstruction of sea-surface temperature, salinity, and sea-ice cover in the northern North Atlantic during the last glacial maximum based on dinocyst assemblages. Canadian Journal of Earth Sciences, 37(5):725–750.
- ↑ Alley, R.B., 2000. The Younger Dryas cold interval as viewed from central Greenland. Quaternary Science Reviews, 19:213–226.– Peteet, D.M. (ed.), 1993. Global Younger Dryas? Quaternary Science Reviews, 12(5):277–356.– Peteet, D.M. (ed.), 1995. Global Younger Dryas Vol. 2, Quaternary Science Reviews, 14(9):811–958.