Factors affecting surface ultraviolet radiation levels in the Arctic
This is Section 5.4 of the Arctic Climate Impact Assessment
Lead Authors: Betsy Weatherhead, Aapo Tanskanen, Amy Stevermer; Contributing Authors: Signe Bech Andersen, Antti Arola, John Austin, Germar Bernhard, Howard Browman,Vitali Fioletov,Volker Grewe, Jay Herman, Weine Josefsson, Arve Kylling, Esko Kyrö, Anders Lindfors, Drew Shindell, Petteri Taalas, David Tarasick; Consulting Authors: Valery Dorokhov, Bjorn Johnsen, Jussi Kaurola, Rigel Kivi, Nikolay Krotkov, Kaisa Lakkala, Jacqueline Lenoble, David Sliney
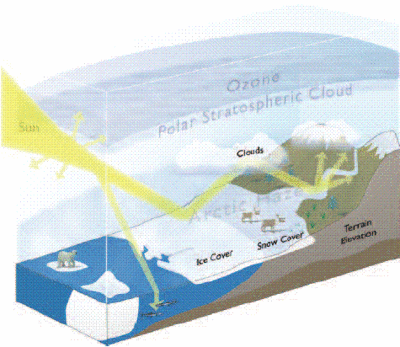
The factors that affect UV radiation levels in the Arctic are generally well established[1], and are illustrated in Fig. 5.5. Atmospheric ozone levels, solar zenith angle, clouds, aerosols, and altitude are all major factors affecting UV radiation levels reaching the surface of the earth. In the Arctic, snow and ice cover add further complexity to the estimation of UV radiation exposure. When UV radiation passes through the atmosphere (Atmosphere layers) it is partially absorbed by ozone, and scattered by air molecules, aerosol particles, and clouds. Attenuation of UV-B radiation as it passes through the ozone layer is primarily a consequence of the sharp increase in the ozone absorption cross section at shorter wavelengths. The ratio of diffuse to global (direct and diffuse) radiation is greater in the UV than in the visible spectrum, primarily due to the wavelength dependence of Rayleigh scattering. Moreover, the ratio is usually higher in the Arctic than at lower latitudes due to large solar zenith angles and frequent snow cover.
Many of the factors affecting UV radiation have large natural variations, which makes it difficult to discern changes in UV radiation levels that result from ozone depletion. Furthermore, the factors are not independent but interact in complex ways. For example, enhancement of surface UV irradiance by multiple scattering depends on both surface albedo and cloud conditions. These features make polar regions, including the Arctic, unique and complex in terms of their UV radiation environments.
Table 5.1 summarizes the factors that affect surface UV radiation levels in the Arctic.
Contents
Extraterrestrial Solar Spectrum (5.4.1)
The radiation output of the sun varies over a range of timescales. Over the last century, the largest variation has been the 11-year solar cycle, which can be estimated by the average number of sunspots. The variation in solar irradiance is dependent on wavelength, with greater variability at shorter wavelengths[2]. It has been estimated, using models and data from the Upper Atmosphere Research Satellite Solar Stellar Irradiance Comparison Experiment instrument, that although the total solar irradiance varies by only about 0.1% over the 11-year solar cycle, the amplitude of variation is as high as 8.3% for wavelengths in the 200 nanometer (nm) range and 0.85% for wavelengths in the 300 nm range[3].
Table 5.1. Factors affecting surface UV irradiance in the Arctic. (Source: ACIA) | ||
---|---|---|
Factor | Correlation with UV doses | Summary remarks |
Solar activity | Negative | In the past century, changes in solar activity have caused fluctuations in surface UV irradiance on the order of a few percent. |
Solar zenith angle | Negative | Diurnal and seasonal changes in solar zenith angle depend on latitude. In the Arctic, seasonal variations are extreme while diurnal variations are smaller than those at lower latitudes. |
Atmospheric ozone | Negative | The amount of ozone in the stratosphere directly affects the amount of UV radiation reaching the troposphere and the surface of the earth. |
Cloudiness | Negative/Positive | Thick clouds can attenuate UV radiation reaching the surface of the earth by tens of percent. Multiple reflections between clouds and snow-covered surfaces can lead to increases in surface UV irradiance, also of the order of tens of percent. |
Atmospheric aerosols | Negative | Aerosols can attenuate UV radiation reaching the surface of the earth. |
Altitude | Positive | Estimated changes in erythemal UV irradiance with altitude vary from 7 to 25% per 1000 m altitude gain. |
Surface albedo | Positive | Reflection off snow can increase surface UV doses by more than 50%. |
Snow and ice cover | Negative/Positive | Changes in the extent and duration of snow or ice cover can expose organisms currently shielded from UV radiation. Organisms living above the snow or ice cover will receive lower UV doses as melting snow or ice reduces the surface albedo. |
Water quality | Not applicable | The amount of UV radiation penetrating through water is affected by UV absorbing dissolved organic carbon. Organisms in the near-surface layer experience the greatest exposure to UV radiation. |
Receptor orientation | Not applicable | The UV radiation doses received by a vertical surface (such as eyes or face) in the Arctic can be substantially higher than those that are received by a horizontal surface. |
Fligge and Solanki[4] reconstructed solar spectral irradiance from 1700 to the present using a model of the magnetic features of the surface of the sun. Their results suggest that since the Maunder solar activity minimum in 1700, solar irradiance has increased by approximately 3% at wavelengths shorter than 300 nm. According to Rozema et al.[5], the increased solar activity since 1700 has led to enhanced atmospheric ozone production and reduced surface UV-B irradiance. Thus, while the 11-year solar cycle has only a small effect on surface UV-B irradiance, longer-term variations in solar activity have the potential to affect future UV radiation levels.
The amount of UV radiation reaching the earth also depends on the distance between the earth and the sun. Due to the eccentricity of the orbit of the earth, this distance varies throughout the year. The earth is closest to the sun on 3 January (perihelion) and farthest away on 4 July (aphelion). The difference between the perihelion and aphelion distances is about 3%, and therefore extraterrestrial irradiance is about 7% higher during the austral (Southern Hemisphere) summer than it is during the boreal (Northern Hemisphere) summer.
Solar Zenith Angle (5.4.2)
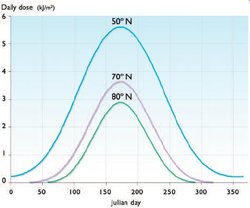
The solar zenith angle (SZA) is the angle between zenith and the position of the sun. Its cosine is approximately inversely proportional to the path length that the direct solar beam has to travel through the atmosphere (Atmosphere layers) to reach the surface of the earth. At large SZAs, when the sun appears low in the sky, atmospheric gases and aerosols absorb more UV radiation owing to the longer path length that photons must travel. Variations in the SZA cause clear diurnal and annual variations in surface UV radiation levels. The SZA is also responsible for most of the latitudinal variation in surface UV radiation levels. The percentage change between summer and winter UV radiation levels is higher in the Arctic than at lower latitudes, while diurnal variations in the SZA are smaller at higher latitudes. In general, SZAs are large in the Arctic and therefore, arctic UV irradiances are typically lower than those at lower latitudes. However, when daily integrated doses are compared, the length of arctic summer days somewhat compensates for the effect of large SZAs. The annual variation of the clear-sky daily erythemal dose at latitudes of 50º, 70º, and 80º N is shown in Fig. 5.6. The values are based on radiative transfer calculations assuming moderate polar ozone levels (300 DU), snow-free conditions with a surface albedo of 0.03, and clear skies. The seasonal variation in erythemal dose is caused solely by the seasonal variation in the SZA.
Ozone Levels (5.4.3)
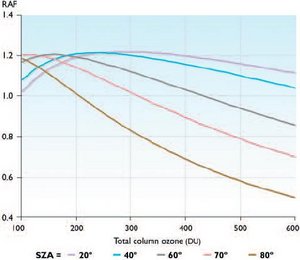
Absorption by ozone causes attenuation of UV-B irradiance. It has been repeatedly demonstrated that a decrease in total column ozone leads to an increase in UV radiation levels[6]. The relationship depends somewhat on the vertical distribution of ozone in the atmosphere. At small SZAs, a redistribution of ozone from the stratosphere to the troposphere leads to a decrease in UV-B radiation levels at the surface[7]. At very large SZAs, this redistribution leads to an increase in UV-B radiation levels[8]. Lapeta et al.[9] and Krzyscin[10] further quantified this effect, and concluded that the erythemally weighted UV dose rate varies by a maximum of 5% owing to changes in the ozone profile.
The change in surface UV irradiance as a result of a change in total column ozone depends highly on the wavelength of the radiation. Traditionally, radiation amplification factors (RAFs) have been used to quantify the change in biologically effective irradiances as a result of a change in total column ozone[11]. These factors can also be used to indicate the sensitivity of a particular UV radiation effect to a change in total column ozone. Values of RAFs depend largely on the biological effect and vary between 0.1 and approximately 2.5[12]. The RAF for the standard erythemal actionspectrum[13] is 1.1 at small SZAs[14], indicating that a 1% decrease in total column ozone leads to a 1.1% increase in erythemal UV radiation. For large changes in total ozone, the relationship is nonlinear, and a more complex relationship is required to estimate the corresponding changes in biologically effective UV radiation[15]. In the Arctic, where SZAs are often large, RAFs should be used with caution due to their pronounced dependence on the SZA and on total column ozone at large SZAs[16]. For example, at an 80º SZA and total column ozone of 300 DU, the erythemal RAF is reduced to approximately 0.8, which is about 27% lower than that for smaller SZAs typical of the mid-latitudes (Fig. 5.7).
Clouds (5.4.4)
The effect of clouds on UV radiation is difficult to quantify because of their complex three-dimensional character and rapid temporal variation. A uniform cloud layer generally leads to a decrease in irradiance at the surface of the earth, because part of the radiation that is reflected upward by the cloud layer escapes into space. However, local surface UV irradiance can be increased if clouds are not obstructing the disk of the sun and additional radiation is reflected from the side of a broken cloud field toward the ground[17]. In meteorology, cloud cover is traditionally measured in “octas”. The sky is divided into eight sectors and the octa number, between zero and eight, is based on the number of observed sectors containing clouds. Bais et al.[18] and Blumthaler et al.[19] showed that when the solar disk is clear of clouds, cloud amounts up to six octas have little effect on irradiance compared to clear-sky situations. Thiel et al.[20] and Josefsson and Landelius[21] have further parameterized the attenuation of UV irradiance as a function of cloud cover and type.
Cloud transmittance of UV radiation depends on wavelength[22]. The maximum transmittance occurs at approximately 315 [[nanometer]s] (nm), although the actual location of this maximum depends on the cloud optical depth, the amount of tropospheric ozone, and the SZA[23]. In general, clouds in the Arctic tend to be optically thinner than clouds at lower latitudes owing to reduced atmospheric water vapor content. When the ground is covered by snow, attenuation of UV radiation by clouds is further diminished owing to multiple scattering between the ground surface and the cloud base[24].
Aerosols (5.4.5)
Aerosols are solid or liquid particles suspended in the atmosphere, found primarily in the lower part of the troposphere. The attenuation of surface UV irradiance by aerosols depends on the aerosol optical depth (AOD), single scattering albedo, asymmetry factor, and aerosol profile. Measurements of AOD are routinely carried out at visible and UV-A wavelengths[25]. The AOD is generally assumed to follow Ångström’s law, which states that AOD is proportional to l-a, where l is wavelength and a is the Ångström coefficient. Converting the AOD measured at longer wavelengths to an AOD value for the UV-B spectrum is not straightforward, however, because a is not easy to measure and is likely to have some wavelength dependence. The single scattering albedo is the ratio of the scattering cross section of the aerosol to its extinction cross section, and is typically greater than 0.95 in relatively unpolluted areas of the Arctic[26].
Episodes of long-range transport of pollutants have been observed in the Arctic. These episodes, combined with the lower rates of particle and gas removal in the cold and stable arctic atmosphere, can lead to a phenomenon called “arctic haze”[27]. Arctic haze events result in increased aerosol concentrations and mostly occur in winter and spring.
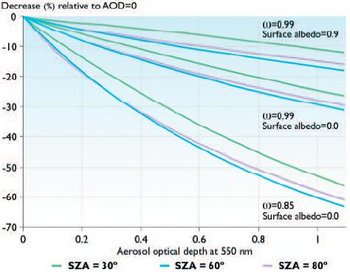
Relatively few studies have addressed the role of aerosols in attenuating solar UV radiation in the Arctic. Wetzel et al.[28] conducted field investigations at Poker Flat, Alaska, and sampled different air mass types originating from sources outside the region. The measured AOD at 368 nm ranged from 0.05 to 0.25, and estimates for the single scattering albedo varied from 0.63 to 0.95, the former being for spring air masses originating from Asia and the latter for cleaner air masses of marine origin. Herber et al.[29] summarized eight years of measurements of AOD at the Koldeway station in Ny Ålesund, Norway, and reported strong arctic haze events, mainly in late winter and spring. The mean AOD at 371 nm during arctic haze conditions was about 0.18, while in the autumn the average AOD was only 0.05. Quinn et al.[30] presented results from three years of simultaneous measurements of aerosol chemical composition and light scattering and absorption at Barrow, Alaska. They found that sulfate concentrations were highest at Barrow and decreased with latitude from Poker Flat to Denali to Homer, suggesting a north–south gradient. Ricard et al.[31] studied the chemical properties of aerosols in northern Finland, and found that, compared to other arctic sites, the aerosols reflect smaller contributions from arctic haze and marine events in winter and larger contributions from biogenic sources in summer. For the range of aerosols sampled at Poker Flat, Alaska, Wetzel et al.[32] found that the attenuation of UV radiation at 305 nm and 368 nm ranged from a few percent up to about 11%.
Figure 5.8 illustrates the decrease in erythemal UV irradiance as a function of AOD, based on theoretical calculations with a radiative transfer model[33]. The figure indicates that in the Arctic, where SZAs tend to be high, the reduction of erythemal UV irradiance by aerosols depends strongly on aerosol properties, including the single scattering albedo, and on surface properties, including surface albedo. In practice, AOD and single scattering albedo cannot be directly translated into UV attenuation, as the asymmetry factor, vertical distribution, and other factors must also be taken into account.
Altitude (5.4.6)
Ultraviolet radiation levels increase with altitude for several reasons. At higher elevations, the atmosphere (Atmosphere layers) is optically thinner, and therefore fewer particles exist to absorb or scatter radiation. Higher elevations also experience a reduced influence from tropospheric ozone or aerosols in the boundary layer. In the Arctic and in mountainous regions, the ground is more likely to be covered by snow at higher altitudes, which leads to higher albedo and increased UV reflectance. Clouds below a mountain summit have a reflective effect similar to snow-covered ground, and will therefore increase UV radiation levels at the summit. In contrast, the same cloud may reduce UV radiation levels in a valley below the mountain. The variation of UV radiation levels with altitude depends on several factors, all of which have different wavelength dependencies; therefore, this variation cannot be expressed by a simple relationship. Changes in erythemal UV irradiance with altitude reported in the literature vary between 7 and 25% per 1000 m of altitude gain[34].
Surface Albedo (5.4.7)
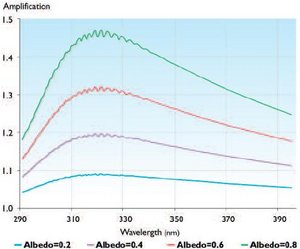
The extent and duration of snow cover in the Arctic has a significant effect on surface UV radiation doses. An increase in surface albedo leads to an increase in downwelling UV radiation, as part of the radiation that is reflected upward is backscattered by air molecules or clouds. Snow is particularly efficient at reflecting UV radiation; multiple reflections between snow-covered ground and clouds, therefore, can lead to a significant increase in surface UV radiation levels compared to a snow-free situation[36].
Surface albedo at UV wavelengths is generally low, except in the presence of snow cover. Blumthaler and Ambach[37] measured erythemally weighted surface albedos for various snow-free surfaces and reported values ranging between 0.01 and 0.11. Spectral measurements by Feister and Grewe[38] and McKenzie and Kotkamp[39] confirm these low values. For snow-covered surfaces, the measurements suggest values ranging from 0.50 to 0.98. In general, dry new snow has the highest albedo, which ranges from 0.90 to 0.98[40]. The albedo of a snow-covered surface depends not only on snow depth and condition, but also on topography, vegetation, and man-made structures[41]. Albedo is an important factor affecting UV radiation levels in the Arctic, where the ground is covered by snow for extensive periods of the year. Figure 5.9 shows the spectral amplification of surface UV irradiance by surface albedo for clear-sky conditions. The figure indicates that snow cover, with an albedo that can be greater than 0.8, can increase erythemal irradiance by up to 60% compared to a snow-free case (albedo 0.2 or less). The amplification is greatest at short UV wavelengths, and thus increases the ratio of UV-B to UV-A radiation.
Scattering in the atmosphere may occur far away from the location of interest; therefore, the ground properties of a large area around the measurement site must be considered. The regionally averaged albedo is often referred to as the “effective albedo”[42], and can be considered the albedo estimate that gives the best agreement between measured and modeled irradiances when used in a radiative transfer model. Three-dimensional radiative transfer models have shown that the area of significance, defined by an increase in UV irradiance of more than 5% when effective albedo is taken into account, can extend more than 40 km around the point of interest[43].
Snow and Ice Cover (5.4.8)
Many arctic ecosystems are shielded from UV radiation for much of the year by snow or ice cover. The transmission of UV radiation through snow or ice depends on wavelength, the thickness of the cover, and the optical properties of the snow or ice. In general, radiation is attenuated by a factor that changes exponentially with the thickness of the snow or ice cover. Shorter wavelengths are more strongly attenuated than longer wavelengths. Field measurements conducted at Alert, Canada, suggest that a 10 cm snow-cover depth reduces the amount of transmitted 321 nm UV radiation by two orders of magnitude[44]. According to Perovich[45], approximately 1.3 meters (m) of white ice would be required to achieve a similar attenuation of transmitted 300 nm UV radiation. It is difficult to project how changes in snow and ice cover will affect the amount of UV radiation to which terrestrial and aquatic life forms in the Arctic are exposed. Observed and projected changes in sea-ice and snow cover are discussed in Sections 6.3 and 6.4 (Factors affecting surface ultraviolet radiation levels in the Arctic), respectively.
Water Quality (5.4.9)
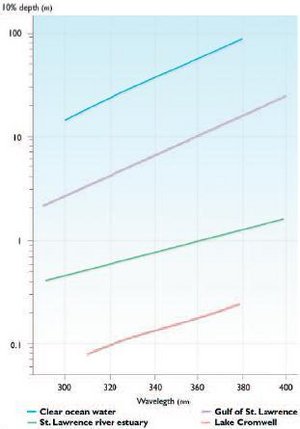
The water quality parameters that are known to affect underwater UV radiation levels are dissolved organic carbon and chlorophyll a[47]. A general optical characterization of water columns is obtained from diffuse attenuation coefficients (Kd(l)), which are calculated from measurements of spectral irradiance at various depths. For comparative purposes, wavelength-specific 10% depths (the depth to which 10% of the below-surface irradiance penetrates) are often derived from the Kd(l) values. It is important to note that the choice of 10% depth is arbitrary and is not based upon any correlation with biological effects. Figure 5.10 shows 10% penetration depths in Lake Cromwell, the St. Lawrence River estuary, and the Gulf of St. Lawrence, Canada. In clear ocean water, 10% of the radiation at longer UV wavelengths can penetrate to a depth of nearly 100 m. In shallower water, this depth may be of the order of only a meter, but organisms living within this 1 m layer would still be at risk. At 310 nm, 10% penetration depths are 20 m for clear ocean water[48]; 1 to 4 m for the Gulf of St. Lawrence and for coastal zones[49]; 0.5 m for estuarine waters; and 0.1 m for Lake Cromwell, Québec[50]. Measurements made in arctic waters suggest that 10% penetration depths are typically less than 5 m[51]. Ultraviolet-A radiation generally reaches greater depths. Organisms residing in the near-surface layer experience the greatest exposure to UV radiation.
Receptor Orientation (5.4.10)
Ultraviolet irradiance has traditionally been measured on a flat, horizontal surface. While this approach has sound physical merit, it does not accurately represent the UV irradiance that reaches many biological receptors. The amount of UV radiation incident on a vertical (as opposed to horizontal) surface has important biological implications, particularly in terms of effects on the eye[52]. Recent studies have explored both the effect of high snow reflectivity[53] and the orientation of the receptor on UV radiation doses. Some investigators have measured the amount of UV radiation incident on a surface oriented perpendicular to the rays of the sun[54] while others have measured the amount incident on a vertical surface[55]. As reported by Webb et al.[56], the relationship between irradiance measured on a horizontal surface and that measured on a vertical surface depends on the orientation of the vertical surface, the SZA, and the wavelength. For wavelengths shorter than 400 nm, Webb et al.[57] found distinct maxima in the vertical to horizontal irradiance ratios during the morning and afternoon. Under cloudless, snow-free conditions, the maximum ratios ranged from 1.4 at 300 nm to 7 at 500 nm. Snow cover, which increases surface albedo, may substantially increase these ratios. For example, in the presence of fresh snow cover and at SZAs greater than 60º, Philipona et al.[58] reported a 65% increase in erythemal UV irradiance on a surface oriented perpendicular to the sun compared to the irradiance observed on a horizontal surface under the same conditions.
Similar results were obtained by Jokela et al.[59], who pointed UV radiometers azimuthally South, North, West, and East to assess UV dose rates on vertical surfaces in Saariselkä, Finland. The results indicated a snow albedo of 0.83, in good agreement with data presented by Blumthaler and Ambach[60]. The ratios of vertical to horizontal dose rates varied from about 0.25 to 1.4, depending on direction and on whether the ground was barren or covered with fresh snow. In general, the observations indicated that spring ozone depletion could greatly increase ocular UV radiation doses because of the significant effect of snow reflection. The measurements by Jokela et al.[61] show that ocular doses of UV radiation in Saariselkä can be higher at the end of April than at any other time of the year. These high doses suggest that the amount of UV radiation incident on the eye when looking toward the horizon can be equivalent or greater than the amount of UV radiation incident on the eye when looking directly upward.
For many biological systems, the actinic flux (the radiation incident at a point) is a more relevant quantity than the horizontal irradiance. Only recently have measurements of the spectral actinic flux become more common[62]. Webb et al.[63] found that the ratio of actinic flux to horizontal irradiance varied between 1.4 and 2.6 for UV wavelengths, and depended on wavelength, SZA, and the optical properties of the atmosphere.
Chapter 5: Ozone and Ultraviolet Radiation
5.1. Introduction (Factors affecting surface ultraviolet radiation levels in the Arctic)
5.2. Factors affecting arctic ozone variability
5.3. Long-term change and variability in ozone levels
5.4. Factors affecting surface ultraviolet radiation levels in the Arctic
5.5. Long-term change and variability in surface UV irradiance
5.6. Future changes in ozone
5.7. Future changes in ultraviolet radiation
5.8. Ozone and Ultraviolet Radiation in the Arctic: Gaps in knowledge, future research, and observational needs
References
Citation
Committee, I. (2012). Factors affecting surface ultraviolet radiation levels in the Arctic. Retrieved from http://editors.eol.org/eoearth/wiki/Factors_affecting_surface_ultraviolet_radiation_levels_in_the_Arctic- ↑ WMO, 2003. Scientific Assessment of Ozone Depletion: 2002. Global Ozone Research and Monitoring Project – Report No. 47. World Meteorological Organization Geneva, 498pp.
- ↑ Solanki, S. and Y. Unruh, 1998. A model of the wavelength dependence of solar irradiance variations. Astronomy and Astrophysics, 329:747–753.
- ↑ Lean, J., 2000. Evolution of the Sun’s spectral irradiance since the Maunder minimum. Journal of Geophysical Research, 27(16):2425–2428.
- ↑ Fligge, M. and S. Solanki, 2000. The solar spectral irradiance since 1700. Journal of Geophysical Research, 27(14):2157–2160.
- ↑ Rozema, J., B. van Geel, L. Björn, J. Lean and S. Madronich, 2002. Toward solving the UV Puzzle. Science, 296:1621–1622.
- ↑ WMO, 2003. Scientific Assessment of Ozone Depletion: 2002. Global Ozone Research and Monitoring Project – Report No. 47. World Meteorological Organization Geneva, 498pp.
- ↑ Brühl, C. and P.J. Crutzen, 1989. On the disproportionate role of tropospheric ozone as a filter against solar UV-B radiation. Geophysical Research Letters, 16(7):703–706.
- ↑ Krotkov, N.A., P.K. Bhartia, J.R. Herman, V. Fioletov and J. Kerr, 1998. Satellite estimation of spectral surface UV irradiance in the presence of tropospheric aerosols - 1. Cloud-free case. Journal of Geophysical Research, 103(D8):8779–8793.
- ↑ Lapeta, B., O. Engelsen and Z. Litynska, 2000. Sensitivity of surface UV radiation and ozone column retrieval to ozone and temperature profiles. Journal of Geophysical Research, 105(D4): 5001–5007.
- ↑ Krzyscin, J.W., 2000. Total ozone influence on the surface UV-B radiation in late spring-summer 1963–1997: An analysis of multiple timescales Journal of Geophysical Research, 105(D4):4993–5000.
- ↑ Booth, C.R. and S. Madronich, 1994. Radiation amplification factors: improved formulation accounts for large increases in ultraviolet radiation associated with Antarctic ozone depletion. In: C.S. Weiler and P.A. Penhale (eds.). Antarctic Research Series, 62, pp. 39–42.-- Van der Leun, J.C., Y.Takizawa and J.D. Longstreth, 1989. Human Health. In: Environmental Effects Panel Report. United Nations Environment Programme, 19pp.-- WMO, 1989. Scientific Assessment of Stratospheric Ozone: 1989. Global Ozone Research and Monitoring Project, Report No. 20, vol. 1, World Meteorological Organization, 387pp.
- ↑ Madronich, S., R.L. McKenzie, L.O. Björn and M.M. Caldwell, 1998. Changes in biologically active radiation reaching the Earth’s surface. Journal of Photochemistry and Photobiology B, 46:5–19.
- ↑ CIE, 1998. Erythema Reference Action Spectrum and Standard Erythema Dose. Joint ISO/CIE Standard, ISO 17166:1999/CIE S007–1998, Commission Internationale de l’Eclairage.
- ↑ Madronich, S., R.L. McKenzie, L.O. Björn and M.M. Caldwell, 1998. Changes in biologically active radiation reaching the Earth’s surface. Journal of Photochemistry and Photobiology B, 46:5–19.
- ↑ Booth, C.R. and S. Madronich, 1994. Radiation amplification factors: improved formulation accounts for large increases in ultraviolet radiation associated with Antarctic ozone depletion. In: C.S. Weiler and P.A. Penhale (eds.). Antarctic Research Series, 62, pp. 39–42.
- ↑ Micheletti, M.I., R.D. Piacentini and S. Madronich, 2003. Sensitivity of biologically active UV radiation to stratospheric ozone changes: effects of action spectrum shape and wavelength range. Photochemistry and Photobiology, 78(5):465–461.
- ↑ Mims, F.M. III and J.E. Frederick, 1994. Cumulus clouds and UV-B. Nature, 371:291.-- Nack, M.L. and A.E.S. Green, 1974. Influence of clouds, haze, and smog on the middle ultraviolet reaching the ground. Applied Optics, 13:2405–2415.
- ↑ Bais, A.F., C.S. Zerefos and C. Meleti, 1993. Spectral measurements of solar UV-B radiation and its relations to total ozone, SO2, and clouds. Journal of Geophysical Research, 98(D3):5199–5204.
- ↑ Blumthaler, M., W. Ambach and M. Salzgeber, 1994a. Effects of cloudiness on global and diffuse UV irradiance in a high-mountain area. Theoretical and Applied Climatology, 50:23–30.
- ↑ Thiel, S., K. Steiner and H.K. Seidlitz, 1997. Modification of global erythemal effective irradiance by clouds. Photochemistry and Photobiology, 65(6):969–973.
- ↑ Josefsson, W. and T. Landelius, 2000. Effect of clouds on UV irradiance: as estimated from cloud amount, cloud type, precipitation, global radiation, and sunshine duration. Journal of Geophysical Research, 105(D4):4927–4935.
- ↑ Frederick, J.E. and C. Erlick, 1997. The attenuation of sunlight by high latitude clouds: spectral dependence and its physical mechanisms. Journal of Atmospheric Sciences, 54:2813–2819.-- Kylling, A., A. Albold and G. Seckmeyer, 1997. Transmittance of a cloud is wavelength-dependent in the UV-range: physical interpretation. Geophysical Research Letters, 24(4):397–400.-- Seckmeyer, G., A. Albold and R. Erb, 1996. Transmittance of a cloud is wavelength-dependent in the UV-range. Geophysical Research Letters, 23(20):2753–2755.
- ↑ Mayer, B., G. Seckmeyer and A. Kylling, 1997. Systematic long-term comparison of spectral UV measurements and UVSPEC modeling results. Journal of Geophysical Research, 102(D7):8755–8768.
- ↑ Nichol, S.E., G. Pfister, G.E. Bodeker, R.L. McKenzie, S.W. Wood and G. Bernhard, 2003. Moderation of cloud reduction of UV in the Antarctic due to high surface albedo. Journal of Applied Meteorology, 42(8):1174–1183.
- ↑ Holben, B.N., T.F. Eck and I. Slutsker, 1998. AERONET-A federated instrument network and data archive for aerosol characterization. Remote Sensing of the Environment, 66(1):1–16.
- ↑ d’Almeida, G.A., P. Koepke and E.P. Shettle, 1991. Atmospheric Aerosols: Global Climatology and Radiative Characteristics. A. Deepak Publishing, 561pp.
- ↑ Shaw, G.E., 1985. On the climatic relevancy of Arctic haze: static energy balance considerations. Tellus B, 37(1):50–52.-- Shaw, G.E., 1995. The Arctic haze phenomenon. Bulletin of the American Meteorological Society, 76:2403–2413.
- ↑ Wetzel, M.A., G.E. Shaw, J.R. Slusser, R.D. Borys and C.F. Cahill, 2003. Physical, chemical, and ultraviolet radiative characteristics of aerosol in central Alaska. Journal of Geophysical Research, 108:10.1029/2002JD003208.
- ↑ Herber, A., L.W. Thomason, H. Gernandt, U. Leiterer, D. Nagel, K.-H. Schulz, J. Kaptur, T. Albrecht and J. Not, 2002. Continuous day and night aerosol optical depth observations in the Arctic between 1991 and 1999. Journal of Geophysical Research, 107(D10): 10.1029/2001JD000536.
- ↑ Quinn, P.K., T.L. Miller, T.S. Bates, J.A. Ogren, E. Andrews and G.E. Shaw, 2002. A 3-year record of simultaneously measured aerosol chemical and optical properties at Barrow, Alaska. Journal of Geophysical Research, 107(D11):4130, doi:10.1029/2001JD001248.
- ↑ Ricard, V., J.-L. Jaffrezo, V.-M. Kerminen, R.E. Hillamo, M. Sillanpää, S. Ruellan, C. Liousse and H. Cashier, 2002. Two years of continuous aerosol measurements in northern Finland. Journal of Geophysical Research, 107(D11):4129–4139.
- ↑ Wetzel, M.A., G.E. Shaw, J.R. Slusser, R.D. Borys and C.F. Cahill, 2003. Physical, chemical, and ultraviolet radiative characteristics of aerosol in central Alaska. Journal of Geophysical Research, 108:10.1029/2002JD003208.
- ↑ Mayer, B., G. Seckmeyer and A. Kylling, 1997. Systematic long-term comparison of spectral UV measurements and UVSPEC modeling results. Journal of Geophysical Research, 102(D7):8755–8768.
- ↑ Blumthaler, M., A.R. Webb, G. Seckmeyer, A.F. Bais, M. Huber and B. Mayer, 1994b. Simultaneous spectroradiometry: a study of solar UV irradiance at two altitudes. Geophysical Research Letters, 21(25):2805–2808.-- Blumthaler, M.,W. Ambach and R. Ellinger, 1997. Increase in solar UV radiation with altitude. Journal of Photochemistry and Photobiology B Biology, 39:130–134.-- Gröbner, J., A. Albold, M. Blumthaler, T. Cabot. A. De la Casiniere, J. Lenoble, T. Martin, D. Masserot, M. Müller, R. Philipona, T. Pichler, E. Pougatch, G. Rengarajan, D. Schmucki, G. Seckmeyer, C. Sergent, M.L. Toure and P.Weihs, 2000. Variability of spectral solar ultraviolet irradiance in an Alpine environment. Journal of Geophysical Research, 105(D22):26,991–27,003.-- McKenzie, R.L., P.V. Johnston, D. Smale, B.A. Bodhaine and S. Madronich, 2001a. Altitude effects on UV spectral irradiance deduced from measurements at Lauder, New Zealand, and at Mauna Loa Observatory, Hawaii. Journal of Geophysical Research, 106(D19):22845–22860.
- ↑ Lenoble, J., 1998. Modeling of the influence of snow reflectance on ultraviolet irradiance for cloudless sky. Applied Optics, 37(12):2441–2447.
- ↑ Kylling, A., A Dahlback and B. Mayer, 2000a. The effect of clouds and surface albedo on UV irradiances at a high latitude site. Geophysical Research Letters, 27(9):1411–1414.
- ↑ Blumthaler, M. and W. Ambach, 1988. Solar UVB-albedo of various surfaces. Photochemistry and Photobiology, 48(1):85–88.
- ↑ Feister, U. and R. Grewe, 1995. Spectral albedo measurements in the UV and visible region over different types of surfaces. Photochemistry and Photobiology, 62(4):736–744.
- ↑ McKenzie, R.L. and M. Kotkamp, 1996. Upwelling UV spectral irradiance and surface albedo measurements at Lauder, New Zealand. Geophysical Research Letters, 23(14):1757–1760.
- ↑ Grenfell, T.C., S.G. Warren and P.C. Mullen, 1994. Reflection of solar radiation by the Antarctic snow surface at ultraviolet, visible, and near-infrared wavelengths. Journal of Geophysical Research, 99:18,669–18,684.
- ↑ Fioletov, V.E., J.B. Kerr, L.J.B. McArthur, D.I. Wardle and T.W. Mathews, 2003. Estimating UV Index climatology over Canada. Journal of Applied Meteorology, 42:417–433.
- ↑ Gröbner, J., A. Albold, M. Blumthaler, T. Cabot. A. De la Casiniere, J. Lenoble, T. Martin, D. Masserot, M. Müller, R. Philipona, T. Pichler, E. Pougatch, G. Rengarajan, D. Schmucki, G. Seckmeyer, C. Sergent, M.L. Toure and P. Weihs, 2000. Variability of spectral solar ultraviolet irradiance in an Alpine environment. Journal of Geophysical Research, 105(D22):26,991–27,003.-- Kylling, A.,T. Persen, B. Mayer and T. Svenøe, 2000b. Determination of an effective spectral surface albedo from ground based global and direct UV irradiance measurements. Journal of Geophysical Research, 105:4949–4959.
- ↑ Degünther, M., R. Meerkoetter, A. Albold and G. Seckmeyer, 1998. Case study on the influence of inhomogeneous surface albedo on UV irradiance. Geophysical Research Letters, 25:3587–3590.-- Lenoble, J., 2000. Influence of the environment reflectance on the ultraviolet zenith radiance for cloudless sky. Applied Optics, 39:4247–4254.-- Ricchiazzi, P.J. and C. Gautier, 1998. Investigation of the effect of surface heterogeneity and topography on the radiation environment of Palmer Station, Antarctica with a hybrid 3-D radiative transfer model. Journal of Geophysical Research, 103, 6161–6176.
- ↑ King, M.D. and W.R. Simpson, 2001. Extinction of UV radiation in Arctic snow at Alert, Canada (82º N). Journal of Geophysical Research, 106(D12):12499–12507.
- ↑ Perovich, D.K., 1993. A theoretical model of ultraviolet light transmission through Antarctic sea ice. Journal of Geophysical Research, 98:22579–22587.
- ↑ Booth, C.R. and J.H. Morrow, 1997. The penetration of UV into natural waters. Photochemistry and Photobiology, 65(2):254–257.-- Kuhn, P., H. Browman and B. McArthur, 1999. Penetration of ultraviolet radiation in the waters of the estuary and Gulf of St. Lawrence. Limnology and Oceanography, 44(3):710–716.-- Scully, N.M. and D.S. Lean, 1994. The attenuation of ultraviolet radiation in temperate lakes. Archiv für Hydrobiologie, 43:135–144.-- Smith, R.C. and K.S. Baker, 1979. Penetration of UV-B and biologically effective dose rates in natural water. Photochemistry and Photobiology, 29(2):311–323.
- ↑ Kuhn, P., H. Browman and B. McArthur, 1999. Penetration of ultraviolet radiation in the waters of the estuary and Gulf of St. Lawrence. Limnology and Oceanography, 44(3):710–716.-- Laurion, I.,W.F. Vincent and S. Lean, 1997. Underwater ultraviolet radiation: Development of spectral models for northern high latitude lakes. Photochemistry and Photobiology, 65(1):107–114.--Morris, D.P., H. Zagarese and C.E. Williamson, 1995. The attenuation of solar UV radiation in lakes and the role of dissolved organic carbon. Limnology and Oceanography, 40(8):1381–1391.--Scully, N.M. and D.S. Lean, 1994. The attenuation of ultraviolet radiation in temperate lakes. Archiv für Hydrobiologie, 43:135–144
- ↑ Smith, R.C. and K.S. Baker, 1979. Penetration of UV-B and biologically effective dose rates in natural water. Photochemistry and Photobiology, 29(2):311–323.
- ↑ Booth, C.R. and J.H. Morrow, 1997. The penetration of UV into natural waters. Photochemistry and Photobiology, 65(2):254–257.-- Kuhn, P., H. Browman and B. McArthur, 1999. Penetration of ultraviolet radiation in the waters of the estuary and Gulf of St. Lawrence. Limnology and Oceanography, 44(3):710–716.
- ↑ Scully, N.M. and D.S. Lean, 1994.The attenuation of ultraviolet radiation in temperate lakes. Archiv für Hydrobiologie, 43:135–144
- ↑ Aas, E., J. Høkedal, N.K. Hørjerslev, R. Sandvik and E. Saksnaug, 2001. Spectral properties and UV-attenuation in Arctic marine waters. In: D.O. Hessen (ed.). UV-Radiation and Arctic Ecosystems, pp. 23–56. Springer-Verlag.
- ↑ Meyer-Rochow, V.B., 2000. Risks, especially for the eye, emanating from the rise of solar UV-radiation in the Arctic and Antarctic regions. International Journal of Circumpolar Health, 59:38–51.-- Sliney, D.H., 1986. Physical factors in cataractogenesis: ambient ultraviolet radiation and temperature. Investigative Ophthalmology and Visual Science, 27:781–790.-- Sliney, D.H., 1987. Estimating the solar ultraviolet radiation exposure to an intraocular lens patient. Journal of Cataract and Refractive Surgery, 13:269–301.
- ↑ McKenzie, R.L., K.J. Paulin and S. Madronich, 1998. Effects of snow cover on UV irradiance and surface albedo: a case study. Journal of Geophysical Research, 103(D22):28785–28792.-- Schmucki, D., S. Voigt, R. Philipona, C. Frohlich, J. Lenoble, A. Ohmura and C. Wehrli, 2001. Effective albedo derived from UV measurements in the Swiss Alps. Journal of Geophysical Research, 106(D6):5369–5383.
- ↑ Philipona, R., A. Schilling and D. Schmucki, 2001. Albedo-enhanced maximum UV irradiance measured on a surface oriented normal to the sun. Photochemistry and Photobiology, 73(4):366–369.
- ↑ Jokela, K., K. Leszczynski and R. Visuri, 1993. Effects of Arctic ozone depletion and snow on UV exposure in Finland. Photochemistry and Photobiology, 58(4):559–566.-- Weatherhead, E.C., G.C. Reinsel, G.C. Tiao, J.E. Frederick, X.L. Meng, D. Choi, W.K. Cheang, T. Keller, J. DeLuisi, D.Wuebbles, J. Kerr and A.J. Miller, 1998. Factors affecting the detection of trends: statistical considerations and applications to environmental data. Journal of Geophysical Research, 103(D14):17,149–17,161.-- Webb, A.R., P. Weihs and M. Blumthaler, 1999. Spectral UV irradiance on vertical surfaces: a case study. Photochemistry and Photobiology, 69:464–470.
- ↑ Webb, A.R., P. Weihs and M. Blumthaler, 1999. Spectral UV irradiance on vertical surfaces: a case study. Photochemistry and Photobiology, 69:464–470.
- ↑ Webb, A.R., P. Weihs and M. Blumthaler, 1999. Spectral UV irradiance on vertical surfaces: a case study. Photochemistry and Photobiology, 69:464–470.
- ↑ Philipona, R., A. Schilling and D. Schmucki, 2001. Albedo-enhanced maximum UV irradiance measured on a surface oriented normal to the sun. Photochemistry and Photobiology, 73(4):366–369.
- ↑ Jokela, K., K. Leszczynski and R.Visuri, 1993. Effects of Arctic ozone depletion and snow on UV exposure in Finland. Photochemistry and Photobiology, 58(4):559–566.
- ↑ Blumthaler, M. and W. Ambach, 1988. Solar UVB-albedo of various surfaces. Photochemistry and Photobiology, 48(1):85–88.
- ↑ Jokela, K., K. Leszczynski and R. Visuri, 1993. Effects of Arctic ozone depletion and snow on UV exposure in Finland. Photochemistry and Photobiology, 58(4):559–566.
- ↑ Hofzumahaus, A., A. Kraus and M. Muller, 1999. Solar actinic flux spectroradiometry: a technique for measuring photolysis frequency in the atmosphere. Applied Optics, 38(21):4443–4460.-- Webb, A.R., A.F. Bais and M. Blumthaler, 2002. Measuring spectral actinic flux and irradiance: experimental results from the Actinic Flux Determination from Measurements of Irradiance (ADMIRA) project. Journal of Atmospheric and Oceanic Technology, 19(7):1049–1062.
- ↑ Webb, A.R., A.F. Bais and M. Blumthaler, 2002. Measuring spectral actinic flux and irradiance: experimental results from the Actinic Flux Determination from Measurements of Irradiance (ADMIRA) project. Journal of Atmospheric and Oceanic Technology, 19(7):1049–1062.