Effects of changes in climate and UV radiation levels on function of arctic ecosystems in the short and long term
This is Section 7.4.2 of the Arctic Climate Impact Assessment
Lead Author: Terry V. Callaghan; Contributing Authors: Lars Olof Björn, F. Stuart Chapin III,Yuri Chernov,Torben R. Christensen, Brian Huntley, Rolf Ims, Margareta Johansson, Dyanna Jolly Riedlinger, Sven Jonasson, Nadya Matveyeva,Walter Oechel, Nicolai Panikov, Gus Shaver; Consulting Authors: Josef Elster, Heikki Henttonen, Ingibjörg S. Jónsdóttir, Kari Laine, Sibyll Schaphoff, Stephen Sitch, Erja Taulavuori, Kari Taulavuori, Christoph Zöckler
Contents
Biogeochemical cycling: dynamics of carbon and nutrients
Arctic ecosystems are characterized by low primary productivity, low element inputs, and slow element cycling, yet they tend to accumulate organic matter, carbon (C), and other elements because decomposition and mineralization processes are even more strongly limited than productivity by the arctic environment, particularly the cold, wet soil environment[1]. Because of this slow decomposition, the total C and element stocks of wet and moist arctic tundra frequently equal and may exceed the stocks of the same elements in the much more productive systems of temperate and even tropical latitudes (Table 7.9).
Low-arctic sites with warmer and dryer soils, and extremely unproductive high-arctic polar deserts and semi-deserts, have smaller accumulations of organic matter (Table 7.10). Most of the organic matter and element accumulation occurs in soils, while large accumulations of biomass are limited by a lack of tall woody plant forms such as trees; by selection for slow-growing, low, compact plant forms; and by low productivity and low availability of soil-available elements such as nitrogen (N) or phosphorus (P). Typically, the majority of the biomass consists of roots and belowground stems, with aboveground plant mass accounting for less than one-third, and sometimes only 5 to 10%, of the total.
In addition to the large C stocks within the seasonally thawed active layer of the soil (Table 7.10), an equally large pool of organic C may be held in the upper permafrost, within 1 to 2 meters (m) of the surface[2]. While these frozen C stocks are not actively involved in C cycling on a seasonal or yearly basis, in the long term, they represent an important C sink, and they are likely to be of particular importance if climate change leads to greater soil thawing or to loss of permafrost (Section 6.6.1.3 (Effects of changes in climate and UV radiation levels on function of arctic ecosystems in the short and long term)).
The largest body of information on organic matter, C, and nutrient budgets of a wide range of arctic ecosystems comes from the IBP Tundra Biome program, which took place during the late 1960s and early 1970s[3]. Since then, research on arctic element cycling has tended to focus on controls over individual biogeochemical processes rather than on comparisons of overall budgets and element stocks. The recent surge of interest in climate change and feedbacks from the Arctic to the globe has highlighted the relevance and utility of those earlier studies, particularly as currently only a few sites are being studied at the whole-system level.
Microbes in arctic [[soil]s] contain only one or a few percent of the ecosystem C pool. However, the proportions of ecosystem N and P are appreciably higher due to high concentrations of N and P in the microbial tissue compared to the concentrations in plants and soil organic matter[4]. As a proportion of the total soil organic matter, microbial biomass and nutrient content are similar to ecosystems outside the Arctic, but as a proportion of the total organic matter in the ecosystem (soil plus vegetation) microbial biomass and nutrient content are high in comparison with other systems due to the relatively small vegetation component in the Arctic. Data from various arctic and subarctic sites have shown that microbes commonly contain appreciably less C, slightly less or comparable amounts of N, and much higher amounts of P than the entire plant biomass[5].
Nutrient mineralization rates are low, however: typically ten-fold lower than in the boreal region. The low rate is mainly due to low soil temperatures, and it leads to low supply rates of nutrients to the plant-available pool and nutrient-constrained plant productivity in most arctic ecosystems[6]. The combination of low mineralization rates and high proportions of nutrients in microbes compared to plants leads to possible competition for nutrients between microbes and plants during periods of rapid microbial growth[7]. However, microbes are likely to also release a pulse of nutrients during periods of population decline when the cells are lysed and nutrients are leached[8]. To project microbial effects on nutrient-constrained plant productivity as a result of environmental changes, it is essential to understand not only how the microbial processing rate of organic matter will change, but also the controls on microbial population sizes and how changes in the populations affect nutrient cycling and interact with plant processes (see also Section 7.4.1.4 (Effects of changes in climate and UV radiation levels on function of arctic ecosystems in the short and long term)).
Table 7.9. Average carbon (C) pools and total C in arctic and alpine tundra, the neighboring boreal zone, and all terrestrial ecosystems. The soil pools do not include organic C in permafrost beneath the seasonally thawed active layer (from Jonasson et al.,[9] after data in McGuire et al.[10]). | |||||||
---|---|---|---|---|---|---|---|
Area (106 km2) | Soil (g C/m2) | Vegetation (g C/m2) | Soil:Vegetation ratio | Total C (1012 kg) | |||
Soil | Vegetation | Soil and vegetation | |||||
Arctic and alpine tundra | 10.5 | 9200 | 550 | 17 | 96 | 5.7 | 102 |
Boreal woodlandsa | 6.5 | 11750 | 4150 | 2.8 | 76 | 27 | 103 |
Boreal forest | 12.5 | 11000 | 9450 | 1.2 | 138 | 118 | 256 |
Global terrestrial | 130.3 | 5900 | 7150 | 0.8 | 772 | 930 | 1702 |
acomparable to forest tundra |
Spatial variability
Although the productivity of the most productive tundra may rival that of highly productive shrub and marsh systems at lower latitudes, most arctic systems lie at the low end of the global productivity range. What is striking is the wide range of variation (about three orders of magnitude) in net primary production (NPP) and standing stocks of organic matter in [[soil]s] and vegetation within the Arctic (Table 7.10). In general, productivity and organic matter stocks decrease with temperature and precipitation from south to north, but local variation in productivity in relation to topography is dramatic (often 10- to 100-fold). Among the most important correlates of topographic variation in productivity are the duration and depth of winter snow cover and degree of protection from winter wind damage, as well as variation in soil moisture, soil thaw, and soil temperature. Local variation in these factors can be nearly as great as that across a wide range of latitudes[11]. Local variation in productivity is also associated with dramatic shifts in the relative abundance of plant functional types including both vascular and nonvascular plants (Sections 7.3 and 7.4.1 (Effects of changes in climate and UV radiation levels on function of arctic ecosystems in the short and long term)). Because of this dramatic local variability, primary production and organic matter accumulation are distributed in a mosaic fashion across the Arctic, with a higher frequency of more productive sites (usually wet or moist lowlands) at lower latitudes.
Table 7.10. Soil organic matter, plant biomass, and net primary production (NPP) in the primary arctic ecosystem types (after Jonasson et al.[12] based on data from Bliss and Matveyeva[13] and Oechel and Billings.[14]) | |||||||
---|---|---|---|---|---|---|---|
Soil organic matter (g/m2) | Vegetation biomass (g/m2) | NPP (g/m2/yr) | Soil:Vegetation Ratio | Soil:NPP Ratio (yr) | Vegetation:NPP Ratio (yr) | Percentage of total area | |
High Arctic | |||||||
Polar desert | 20 | 2 | 1 | 10 | 20 | 2.0 | 15 |
Semi-desert | 1030 | 250 | 35 | 4.1 | 29 | 7.1 | 8 |
Wet sedge/mire | 21000 | 750 | 140 | 28 | 150 | 5.4 | 2 |
Low Arctic | |||||||
Semi-desert | 9200 | 290 | 45 | 32 | 204 | 6.4 | 6 |
Low shrub | 3800 | 770 | 375 | 4.9 | 10 | 2.1 | 23 |
Wet sedge/mire | 38750 | 959 | 220 | 40 | 176 | 4.3 | 16 |
Tall shrub | 400 | 2600 | 1000 | 0.21 | 0.4 | 2.6 | 3 |
Tussock/sedge dwarf shrub | 29000 | 3330 | 225 | 8.7 | 129 | 16 | 17 |
asource data for this value, which is surprisingly low, have not been found |
The spatial distribution of productivity and organic matter in the Arctic is broadly predictable in relation to temperature, soil moisture, and other soil factors such as pH, topography, and snow cover[15]. The proximate controls on C cycling in these ecosystems, however, are much more closely tied to the inputs and turnover of other elements, especially N and P[16]. Because N and P inputs (by deposition, fixation, or weathering) are low, even where rapid photosynthesis is possible C cannot be stored in organic matter any faster than the rate of N or P accumulation. Thus, for example, in the Canadian High Arctic where a large portion of the surface is bare ground, C fixation and accumulation is closely tied to low, wet areas where anaerobic soil conditions favor N fixation[17]. In other arctic systems, such as Alaskan wet and moist tundras, the total amounts of N and P in soil organic matter may be very high but, due to slow rates of decomposition, the availability of these elements to plants is low, leading to low productivity despite high element and soil organic matter stocks[18].
Temporal variability
Interannual variation in biogeochemical cycles has received little attention in the Arctic, although a few multi-year records of ecosystem C exchange, N deposition, and C and N losses at the watershed or catchment level do exist[19]. Clearly, the trend over the Holocene, at least, has been one of overall accumulation of elements in organic matter since the loss of the glacial ice cover, but the variation in rates of accumulation (or loss) at the scale of years to decades is particularly poorly understood.
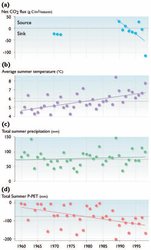
The net C balance of arctic ecosystems, as in any terrestrial ecosystem, may be positive or negative depending on the timescale over which it is measured and the environmental conditions during the measurement period. This dynamic balance is called net ecosystem production (NEPa) and is defined as the difference between two large, opposing fluxes: gross ecosystem production (GEP, or gross ecosystem photosynthesis) and ecosystem respiration (RE), both measured in units of C (mass or moles) per unit area and time. Ecosystem respiration has two major components, RA (autotrophic or plant respiration) and RH (heterotrophic or animal plus microbial respiration). Each of these components of NEP has different relationships to current temperature, moisture, and light conditions. Because they are measured at the whole-system level, all three components are also a function of the current functional mass or surface area of the organisms as well as their current nutritional status. Thus, for example, even though longterm NEP must be positive for the large C accumulations in tundra ecosystems to occur, on a daily or seasonal basis NEP swings from strongly negative (at "night", even under the midnight sun, or in winter) to strongly positive (at midday in midsummer). These daily and seasonal fluctuations have been measured at an increasing number of arctic sites in recent years[21].
Carbon balance may also vary sharply among years, and may be either positive or negative on an annual basis. Recent work in Alaska[22] indicates that although C was accumulating in wet and moist tundras in the 1960s and 1970s (i.e., "negative" C balance), during much of the 1980s and 1990s there was a net loss of C from these ecosystems in both winter and summer (i.e., "positive" C balance). In the late 1990s, the summer C balances changed again so that the ecosystems were net sinks, but it is not yet clear whether the C balances for the full year have returned to net C accumulation (Fig. 7.26; see also Section 7.5.1.1 (Effects of changes in climate and UV radiation levels on function of arctic ecosystems in the short and long term)). It is also unclear whether the shifts in NEP (from C sink to C source) that have occurred over the past 40 years are related in any direct way to weather, because the entire period has been one of general warming in northern Alaska (Section 7.5 (Effects of changes in climate and UV radiation levels on function of arctic ecosystems in the short and long term)). Modeling studies (e.g., [23]) suggest that in the short term (within one or a few years) the response of RE (both RA and RH) to temperature is more rapid than the response of GEP, leading to a short-term loss of C with warming. In the long term, however, the interaction between temperature and soil nutrient availability might increase GEP sufficiently to cause an eventual return to net C accumulation. There is also evidence from manipulation experiments[24] and latitudinal gradients[25] that increases in air temperature can result in soil cooling after long periods, as higher air temperatures lead to increased leaf area indices that intercept a greater proportion of incoming radiation before it reaches the soil surface, thereby leading to soil cooling.
Species and functional type composition of the vegetation are keys to long-term change in productivity, because of differences in nutrient use and allocation, canopy structure, phenology, and relative growth rates among plants[26]. Large differences exist, for example, in the rate at which tundra plants can respond to changes in weather and climate, due to differences in allocation to stems versus leaves or to secondary chemistry versus new growth[27], in the ability to add new meristems[28], and in the constraints on the amount of growth that can be achieved by a single meristem within a single year (i.e., determinate versus indeterminate growth). Species and functional types also differ in their growth phenology and thus in their ability to take advantage of a change in the timing and duration of the growing season. For example, moss-dominated ecosystems in Iceland have limited ability to respond to climate change without a complete change to a vascular plant-dominated community[29], whereas shrubs and small trees already present in sheltered, moist depressions on the North Slope of Alaska seem to be already expanding their distribution[30] and therefore productivity.
The chemical composition of primary productivity (leaves versus wood, secondary chemistry, species composition) is important as a long-term feedback to productivity and its responsiveness to climate change. It is also important in terms of both animal community composition and secondary (herbivore) productivity. [[C]arbon] to nitrogen ratios; lignin and protein content; and tannin, resin, and phenolic content are all important in determining forage quality ([[Section 7.4.1.4 (Effects of changes in climate and UV radiation levels on function of arctic ecosystems in the short and long term)]2]) and the susceptibility of plant litter to decomposition, and thus the remineralization of essential limiting nutrients like N and P[33].
Despite the critical importance of NPP together with NEP and the considerable research already conducted on these parameters, additional field measurements and focused process studies are needed to resolve issues relating to the different methodologies used for measuring NPP and NEP[34]. Results from different methodologies also need to be reconciled (Section 7.7.1.1 (Effects of changes in climate and UV radiation levels on function of arctic ecosystems in the short and long term)).
Budgets of N and P were developed for several arctic sites during the IBP studies 30 years ago[35] but complete documentation of inputs and outputs of any element other than C has not been attempted since then for any arctic site. Part of the problem is that individual N and P inputs and outputs in arctic ecosystems, such as N fixation, N deposition, denitrification, rock weathering, or losses in streamflow (see next subsection), are even smaller than the amounts annually recycled by mineralization of organic matter[36] (Fig. 7.27), except perhaps in the high Arctic[37]. Thus, very long-term records are needed to evaluate the significance of interannual variation in N and P budgets, while most studies of the component processes last only one to three years.
Inputs/outputs, primary production, and net ecosystem production
The dominant C input to arctic ecosystems is from photosynthesis in vascular and nonvascular plants, which in total comprises GEP. The relative (apparent) importance of various controls on primary production differs depending on the level (leaf, canopy, or whole vegetation) and timescale (daily, seasonal, or decadal) of examination[38]. Carbon inputs at the leaf level are clearly limited in the short term by generally low irradiance and consequent low temperatures during usually short, and late, growing seasons (Fig. 7.8), despite a wide range of specific photosynthesis-related adaptations to the arctic environment (Section 7.3 (Effects of changes in climate and UV radiation levels on function of arctic ecosystems in the short and long term)). Photosynthesis in arctic plants is also often sensitive to changes in carbon dioxide (CO2) concentrations (in the short term), moisture conditions, and snow (UV radiation effects are variable and comparatively small). Although arctic plants in general are well adapted to the arctic climate, there is considerable variation in the responses of photosynthesis to microclimate among plant functional types. In the longer term and at the level of whole vegetation canopies, however, C inputs are limited by generally low canopy leaf areas, leaf phenology and duration, and light interception[39]. Canopy leaf area is low because low soil nutrient availability, particularly N, limits the ability of the vegetation to develop a large, photosynthetically efficient leaf area[40], and it also limits the ability of the vegetation to use newly fixed C for new growth, because growth requires adequate supplies of multiple elements in addition to C[41]. It is also low because of the low stature of the vegetation, which prevents development of a multilayered canopy. Other environmental factors such as wind and soil disturbance also limit C gain. Storage of photosynthate and nutrients acquired in previous years plays a key role in determining the current year’s productivity[42].
[[C]arbon] outputs from arctic ecosystems occur via a wider array of processes and are regulated very differently from C inputs (Section 7.4.2.2 (Effects of changes in climate and UV radiation levels on function of arctic ecosystems in the short and long term)). The dominant form of C loss is as CO2, produced by both plants and soil biota. Autotrophic or plant respiration (RA) typically accounts for about half of GEP on an annual basis[43] but follows a very different seasonal and daily pattern (discussed previously).
Heterotrophic respiration (RH), mostly by soil organisms, accounts for most of the other half of GEP, although in the long term the sum of RA and RH must be slightly less than GEP if C is to accumulate in soil organic matter. Heterotrophic respiration produces both CO2 and methane (CH4), the latter produced anaerobically in wet soils ([[Section 7.4.2.2 (Effects of changes in climate and UV radiation levels on function of arctic ecosystems in the short and long term)]2]). Much of the CH4 produced in arctic soils is oxidized to CO2 before it reaches the atmosphere; net CH4 emissions thus are normally only a fraction of CO2 emissions from arctic soils (less than 5%), but CH4 is a much more powerful greenhouse gas than CO2. Other aspects of C balance are important yet difficult to quantify. Examples are plant root respiration, the sloughing of dead material from roots, root exudation, and the growth and respiration of microorganisms intimately associated with plant roots.
Most of the respiratory CO2 and CH4 losses from arctic systems move directly to the atmosphere. Significant fractions of these gases, however, travel in dissolved forms in soil water, eventually reaching streams and lakes where they are released to the atmosphere[44]. In addition, soil and surface waters contain significant amounts of dissolved organic forms of C, much of which is eventually consumed by aquatic microbes, producing more CO2 (Chapter 8 (Effects of changes in climate and UV radiation levels on function of arctic ecosystems in the short and long term)). Together, these losses to aquatic systems may add up to a significant component of the net C balance of arctic systems. Synoptic, simultaneous analysis of aquatic C losses at the same time, place, and scale as direct atmospheric exchanges has not been completed, but estimates of aquatic C losses suggest that these may equal as much as 20 to 30% of GEP.
Winter CO2 losses are a second major gap in the understanding of C losses from arctic ecosystems. Although winter CO2 losses have long been recognized[45], more recent research indicates that these losses are greater than was previously thought and may be the product of significant respiratory activity during the winter[46] (Section 7.3.2.3 (Effects of changes in climate and UV radiation levels on function of arctic ecosystems in the short and long term)), when recently fixed C is respired[47].
There are few studies of inputs and outputs of N in the Arctic, largely because early work suggested that they were small relative to standing N stocks and internal recycling, and thus were less important, at least on a short-term basis[48]. In the long term (several decades or more), however, understanding of N inputs and outputs is essential to understanding how the total pool sizes of N change over time. Changes in standing stocks of N are closely tied to the accumulation or loss of organic matter and C in the Arctic[49].
Nitrogen enters arctic ecosystems through atmospheric deposition and microbially mediated N fixation (Fig. 7.27). Nitrogen deposition rates are low in the Arctic relative to other parts of the world, mostly because the atmosphere is cold enough that it cannot hold the high concentrations of N species such as nitrate (NO3) that are deposited at lower latitudes. Thus, N deposition can account for only about 5% or less of the annual plant N uptake requirement in Alaskan wet-sedge tundra[50], although this might increase with increased industrial activity at lower latitudes. In regions such as northern Scandinavia that are subject to N deposition from lower-latitude anthropogenic sources, however, N deposition may be greater than 0.1 grams per square-meter per year (g/m2/yr), which if continued for many years is sufficient to affect plant growth and productivity[51]. Nitrogen fixation rates are usually assumed to be of similar magnitude, although the only relatively recent studies[52] indicate that, at least in the high Arctic, N fixation might account for more than 10% of plant requirements with the remaining 90% supplied by recycling from the [[soil]s].
[[N]itrogen] losses are also poorly understood. There have been no recent, published studies of denitrification in the Arctic; although anaerobic soils might be expected to have high potential for denitrification, the generally low rates of NO3 production in tundra soils suggest that this is also a small component of the annual N budget. Possible spring losses of N in the form of nitrous oxide (N2O) have been suggested[53] but not yet verified in the Arctic. Nitrogen losses in streams have been monitored (Monitoring) at several locations, and are of roughly the same magnitude as N deposition[54].
Responses to climate change
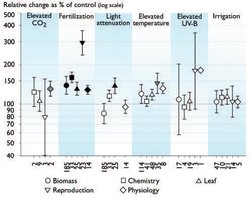
Responses of element cycles in arctic ecosystems to climate change factors have been studied in multi-year manipulation experiments in several contrasting ecosystem types[56] (Fig. 7.28). These experiments include manipulations of air temperature, CO2 concentration, light, water (both excess and deficit), nutrients, and UV-B radiation levels. One common observation from these experiments is that although short-term responses to single factors like changing CO2 concentrations or temperature increases are measurable and often significant, these responses are often not sustained due to other limitations. A general conclusion is that nutrient limitation dominates the multi-year responses and is linked to changes in other factors (e.g., temperature and water) through their indirect effects on nutrient mineralization and availability to plants. In wet systems, water-table depth and soil drainage are critical variables limiting nutrient turnover in the soil; increases in C turnover in these systems are not linked to increases in C accumulation because increased C accumulation requires increased N and/or P supply[57]. A large pool of nutrients exists in organic matter, and may drive large changes in organic matter stocks if the nutrients can be mineralized and not leached from the system. Similarly, short-term increases in photosynthesis and growth in response to high CO2 concentrations are often not sustained due to nutrient limitation[58] ([[Section 7.3 (Effects of changes in climate and UV radiation levels on function of arctic ecosystems in the short and long term)]2]).
The results of several manipulation experiments indicate that nutrient mineralization stimulated by increased soil temperatures is unlikely to be sustained in the long term. Soil temperature increases cause an immediate increase in soil respiration in laboratory studies, but few arctic field studies have shown increased mineralization rates in response to increased air temperatures (but see Schmidt et al.[59]) and longer-term field studies show an acclimation to increased soil temperature[60]. Some studies have shown soil cooling in response to increasing air temperatures in experiments[61] and along latitudinal gradients[62]. Higher air temperatures stimulate leaf area development[63] and a greater leaf area index would be expected to intercept thermal radiation before it reaches the soil, leading to soil cooling. In addition, organic matter in deeper soil profiles is less responsive to temperature increases than that in surface layers[64], again suggesting that any temperature-induced mineralization is likely to be transitory.
Overall, however, multi-year experiments suggest that the arctic ecosystems most responsive to climate change are very likely to be those in which the environmental change is linked to a large change in nutrient inputs or soil nutrient turnover, and/or large changes in leaching or erosional losses of soil-available nutrients (Fig. 7.27). The effects of UV radiation on overall organic matter cycling are generally unknown, but not unimportant. Recent work by Niemi et al.[65] showed that increased UV-B radiation decreased CH4 emissions from a peatland in northern Finland, while three studies show UV-B radiation effects on Sphagnum growth[66] with potential implications for C sequestration. Long-term responses of biogeochemical cycling to increased CO2 concentrations and UV-B radiation levels are small in magnitude but are likely to lead to longer-term changes in biogeochemical cycling and ecosystem structure (Sections 7.3.3.3 and 7.4.1.2 (Effects of changes in climate and UV radiation levels on function of arctic ecosystems in the short and long term)). However, most of the current understanding of UV radiation responses is based on species- and tissue-level research.
Biodiversity and species effects on biogeochemistry
It is important to determine if species or growth form composition of the vegetation have any impact on biogeochemistry of arctic ecosystems, or if biogeochemistry is largely regulated by climate and resource availability irrespective of species composition. Although only partial answers to this question are currently available, there are at least five main mechanisms by which species composition are likely to have important consequences for biogeochemistry. These are:
- Species composition is likely to affect the rate of change in ecosystems in response to environmental change, through differences in species growth, reproduction, and dispersal rate potential (e.g., Bret-Harte et al.[67]).
- Species are likely to affect nutrient availability and C cycling through differences in the turnover of elements in their living tissues and in the decomposability of their dead parts[68].
- Species are likely to affect element accumulations in living plants through differences in their biomass allocation patterns and in their biomass element concentrations and element ratios[69].
- Species are likely to differ in their effects on snow accumulation and snowmelt, surface energy balance, and soil temperature regimes, with important feedbacks to element cycles[70].
- Physiological mechanisms, for example, wetland species that act as conduits for CH4 transport from the soil to the atmosphere[71] (Fig. 7.29).
All five of these species effects have been documented in arctic systems, although it is often uncertain how to scale up from small experimental communities to larger units of the landscape.
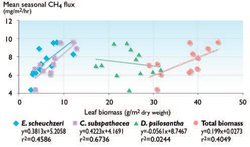
Species richness or diversity (Species diversity) itself is likely to also affect the biogeochemistry of arctic ecosystems, although the magnitude of the effect is hard to judge. There is a weak positive correlation between productivity and vascular species richness in arctic vegetation, but, like most vegetation, richness declines when productivity is increased artificially by fertilizer addition or other disturbance[73]. Recent evidence suggests that arctic plants obtain their N from diverse sources in the soil[74] and that the relative abundance of different species reflects different abilities to acquire the different forms of N[75]. These latter studies suggest that diversity will probably increase productivity in arctic vegetation by increasing total uptake of different forms of a strongly limiting element, N. Partial support for this conclusion comes from experiments involving removal of individual species from arctic vegetation, in which the remaining species failed to increase in abundance[76].
Vascular plants directly affect the substrate availability for methanogens and have the capability to transport gases between the anaerobic parts of soils and the atmosphere[77]. Different vascular plant species have different effects, however, and the vascular plant species composition in wet tundra ecosystems may be a key determinant of the scale of CH4 emissions; for example, Fig. 7.29 shows that the minor constituents of the total vascular plant biomass (Carex and Eriophorum) seem to be “driving” net CH4 emissions from the site, suggesting that shifts in vascular plant species composition alone could lead to significant effects on trace-gas exchange[78]. Changes in species composition per se caused by climate warming and increased UV-B radiation levels are likely to cause a change in CH4 emissions, adding to the direct effect of a changing soil climate on these emissions[79] (see [[Section 7.4.2.2 (Effects of changes in climate and UV radiation levels on function of arctic ecosystems in the short and long term)]3]).
Role of disturbance
Disturbances are expected to increase with climatic warming, mainly through thermokarst (Section 7.5.2 (Effects of changes in climate and UV radiation levels on function of arctic ecosystems in the short and long term)) and possibly also through increased fire in some northern ecosystems and insect pest outbreaks in subarctic forests ([[Section 7.4.1.4 (Effects of changes in climate and UV radiation levels on function of arctic ecosystems in the short and long term)]3]). In general, physical disturbance of arctic ecosystems results in greater soil warming and permafrost thawing, which tend to increase soil organic matter and nutrient turnover. Typically, vegetation productivity increases dramatically although soil respiration also increases. It is not yet clear whether the increased plant growth is sufficient to compensate for losses of soil organic matter. In the long term, however, arctic landscapes should gain organic matter in both [[soil]s] and vegetation on disturbed sites. The timing and trajectory of these changes are key unknowns requiring further research.
Soil processes and controls over trace-gas exchanges
During the last decade, trace-gas production, emissions, and assimilation have attracted considerable attention from the scientific community, for the following reasons:
- most of these gases are “radiatively active” (i.e., they affect the heat balance and contribute to the “greenhouse effect” responsible for climate instability and change);
- the atmospheric concentration (mixing ratios) of these gases underwent remarkably rapid changes after the industrial revolution (e.g., the concentration of atmospheric CH4 has been growing at an annual rate of 0.8 to 1%, which can have a significant impact on the biosphere apart from the greenhouse effect); and
- most trace gases are intermediate or end products/ substrates of key biogeochemical processes, such that monitoring these gas species can be used for early detection of any anomaly in ecosystem functioning.
Table 7.11 lists the major trace gases and their potential impacts on ecosystems, although not all of the listed gases are of primary importance for arctic terrestrial ecosystems. This review is restricted to CO2 (Section 7.4.2.1 (Effects of changes in climate and UV radiation levels on function of arctic ecosystems in the short and long term)), CH4, and N2O.
Soil and ecosystem processes responsible for trace-gas emissions
Trace-gas exchange with the atmosphere occurs through a set of coupled soil/ecosystem processes, including:
- production of substrate(s) for processing by trace gas-producing organisms;
- conversion of substrate(s) to respective gaseous species in parallel with gas consumption (Table 7.12); and
- mass transfer of produced gas to the free atmosphere, which includes three main mechanisms: molecular diffusion, vascular gas transfer (i.e., through plant “conduits”), and ebullition (i.e., bubble formation).
Substrates are formed by one of three processes: decomposition (hydrolytic breakdown of plant litter, oxidation, fermentation); N mineralization, and photosynthesis and photorespiration. However, the starting point for almost all substrates is the primary production of organic matter by plant photosynthesis or (occasionally) bacterial chemosynthesis. There are two main flows of C substrates from plants: plant litter formation with lignocellulose as a main component resistant to microbial breakdown; and the continuous supply of readily available C monomers (root and foliage exudation). The chain of events leading to the formation of immediate precursors of trace gases can be long and intricate[80]. It is worthwhile to note that the most successful model simulations of trace gas emissions include vegetation or primary productivity modules.
Trace-gas transport
There are three main transport mechanisms: molecular diffusion, vascular transport of gas through plant roots, and ebullition. Vascular transport can be described as a diffusion process through plant root aerochyma (parenchyma containing large air spaces typical of emergent and marginal wetland species), which is a continuous network of gas-filled channels. Vascular transport is two or three orders of magnitude more rapid than diffusion in water. Ebullition is probably the most difficult process to simulate and describe mathematically due to its stochastic nature. In northern [[soil]s], ebullition and vascular transport were shown to be the major transport mechanisms, accounting for up to 98% of total CH4 emissions[81].
Environmental controls on methane fluxes
Methane is produced from anaerobic decomposition of organic material in waterlogged, anaerobic parts of the soil. Wet and moist tundra environments are known to be significant contributors to atmospheric CH4[82]. Methane is formed through the microbial process of methanogenesis, which is controlled by a range of factors: most notably temperature, the persistence of anaerobic conditions, gas transport by vascular plants, and the supply of labile organic substrates[83]. Figure 7.30 shows the variety of controls on CH4 formation rates at different spatial and temporal scales. Methane is not only produced but also consumed in the aerobic parts of the soil through the microbial process of methanotrophy, which can even take place in dry soils with the bacteria utilizing atmospheric CH4[84]. Methanotrophy is responsible for the oxidation of approximately 50% of the CH4 produced at depth in the soil and therefore is as important to net CH4 emissions as methanogenesis.The anaerobic process of methanogenesis is much more responsive to temperature than CH4 uptake, so soil warming in the absence of any other changes is very likely to accelerate emissions (the difference between production and consumption), in spite of the simultaneous stimulation of the two opposing processes.
Table 7.11. Trace gases produced in tundra soils and their potential impacts on terrestrial ecosystems. | |||||
---|---|---|---|---|---|
Gas species | Main soil sources | Main soil sinks | Environmental control on "source–sink" balance | Impact a | Reference related to the Arctic |
Carbon dioxide (CO2) | Organic matter decomposition | Photosynthetic uptake; formation of carbonates |
Temperature; moisture; available nutrients |
GHG; productivity of plant community (indirectly) |
[85] |
Methane (CH4) | Methanogenesis (anaerobic decomposition of organic matter) |
Uptake by methanotrophic bacteria |
Temperature; moisture; nutrients; plant community structure | GHG; highly combustible | [86] |
Nitrous oxide (N2O) |
Denitrification; nitrification | Uptake by aerobic and anaerobic soil bacteria |
Temperature; moisture; N [[fertilizer]s] |
GHG | [87] |
Carbon monoxide (CO) |
Anaerobic decomposition of organic matter; airborne pollution | Uptake by carboxydobacteria | Temperature; moisture | Atmospheric photochemical reactions; toxicity | [88] |
Molecular hydrogen (H2) |
Fermentation, Pollution | Uptake by methanogens, acetogens, sulfite reducers, and H2-oxidizing bacteria | Temperature; moisture | Atmospheric photochemical reactions; toxicity | [89]; no specific arctic works |
non-methyl hydrocarbons (terpene and isoprene derivatives) | Plants and microorganisms (bacteria, fungi) | Photooxidation; uptake by aerobic soil bacteria | Temperature; moisture; plant phenology and physiological state |
GHG; ozone and aerosol formation within plant canopy |
[90] |
Methylated halogens (methyl bromide, methyl iodide, etc.) |
Soil affected by oceanic water |
Bacterial decomposition | Soil hydrology; location within landscape relative to oceanic shoreline | Ozone depletion; phytotoxicity | [91] (data for Irish peatland ecosystems) |
Dimethyl sulfide (DMS) | Hydrolysis; uptake as a sulfur source by plants | Soil hydrology and location within landscape relative to oceanic shoreline | Phytotoxicity | [92] | |
Sulfur oxides (SO2; SO) |
Fuel combustion: airborne contamination of soil |
Sulfate and sulfite formation; ion exchange; plant and microbial uptake | Temperature; moisture |
Acid rain; phytotoxicity |
[93] |
Ammonia (NH3) | Airborne soil contamination | Conversion to ammonium (NH4+); ion exchange; plant and microbial uptake | Temperature; moisture | Plant productivity | [94] |
a GHG=greenhouse gas |
Apart from temperature, water regime, and plant cover, methanogenic bacteria are strongly affected by biological interactions within the soil community. Competition with acetogenic and sulfidogenic bacteria for molecular hydrogen (the outcome of which depends on the affinity to hydrogen, the temperature, and density of the various populations) determines the pattern of gas formation not only quantitatively, but also in qualitative terms. For example, ecosystems can be a source of CH4 (if methanogenic bacteria prevail), or hydrogen sulfide and other sulfides (if sulfate-reducing bacteria dominate), or acetic acid (if a large population of acetogens is present).
Early empirical models of northern wetland/tundra CH4 exchanges suggested sensitivity to climate change[95]. A simple mechanistic model of tundra CH4 emissions that included the combined effects of temperature, moisture, and active-layer depth also suggested significant changes in CH4 emissions as a result of climate change[96]. More complex wetland CH4 emission models suggest that winter processes have a strong influence on net annual CH4 emissions[97]. Variations in CH4 emissions at the regional and global scale are driven largely by temperature[98] with the important modulating effects of vascular plant species composition superimposed[99]. An initial warming is, hence, projected to lead to increased CH4 emissions, the scale of which will depend on associated changes in soil moisture conditions and the secondary effects of changes in vegetation composition (Section 7.5.1.2 (Effects of changes in climate and UV radiation levels on function of arctic ecosystems in the short and long term)).
Table 7.12. Examples of soil processes where trace gases are formed or consumed. | ||
---|---|---|
Gas species | Source reaction | Sink reaction |
CO2 | Oxidative decomposition of dead organic matter (OM): OM+O2?CO2+H2O+"ash" Oxidation of root exudates Fermentation: (CH2O)n a? CO2+VOC b+H2 |
sulfur c (S) oxidizing bacteria Photosynthesis (plant and green bacteria): nCO2+nH2O ?nCH2O a +nO2 Soil chemosynthesis (nitrifying, hydrogen-oxidizing, iron (Fe2+)-oxidizing, and sulfur c (S) bacteria) Carbonate formation and leaching |
CO | Fermentation O2-limited oxidation reactions |
Activity of carboxydobacteria: CO + 1/2 O2 ? CO2 Spontaneous chemical oxidation |
CH4 | Methanogenesis: CO2 + H2 ? CH4 or CH3COO-(acetate)? CH4 | Methanotrophy: CH4 ? CO2 + H2O Photochemical reactions with hydroxyl and chlorine radicals |
N2O | Nitrification: NH4+?? 99% NO3- + ? 1% N2O Denitrification NO3- ? N2O ? N2 (molecular nitrogen) |
The N2O is finally converted to stable end products such as NO3- (nitrification, aerobic conditions) or N2 and NH3 (denitrification, anaerobic conditions ) upon completion of the respective processes |
Nitric oxide (NO) | Nitrate reduction NO3- ? NO | NO oxidation |
Isoprene (C5H8) | Secondary metabolic reactions in plants: pyruvate ? ... ? isoprene Fermentation |
Microbial oxidation Formation of phytogenic aerosols and sedimentation |
a carbohydrate; b volatile organic carbon (acetic, propionic, or butyric acid; aldehydes; alcohols; ketones; ethers; etc.); c these bacteria can use several S-containing reduced compounds (S, S2-, SO32-, etc.) as an energy source |
Controls on nitrous oxide fluxes
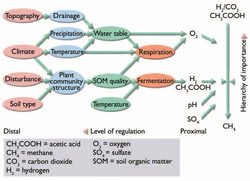
The simulation of N2O emissions requires consideration of the combined N (Nitrogen cycle) and C cycles, because the substrates of denitrification include electron acceptors (NO3) as well as oxidizable C substrates. The ecosystem–atmosphere fluxes of N2O are associated with fundamental transformations of N in the soil, namely the processes of nitrification and denitrification. Very few field studies of N2O fluxes are available from the Arctic[101], but very small releases of N2O are expected from arctic soils due to their general nutrient limitation. However, one issue of potentially great importance in the Arctic is early spring fluxes of N2O. Denitrification has been found to take place even below the freezing point[102]. During freezing and thawing, C is liberated and this may increase denitrification activity in the soil[103]. During the spring thaw of the soil, a significant percentage of annual N2O emissions can take place[104]. The early spring fluxes may also explain the significant N2O production measured in fertilized plots on a subarctic heath[105] on soils that showed no emissions from control plots. Hence, as with CH4, the winter and "shoulder" season processes are generally very important but also the least well understood.
Water and energy balance
Arctic ecosystems exhibit the greatest seasonal changes in energy exchange of any terrestrial ecosystem because of the large changes in albedo from late winter, when snow reflects most incoming radiation, to summer when the ecosystem absorbs most incoming radiation. About 90% of the energy absorbed during summer is transferred to the atmosphere, with the rest transferred to the soil in summer and released to the atmosphere in winter[106]. Consequently, arctic ecosystems have a strong warming effect on the atmosphere during the snow-free season.
Vegetation profoundly influences the water and energy exchange of arctic ecosystems. In general, ecosystems with high soil moisture have greater evapotranspiration than dry ecosystems, as in any climatic zone. Arctic ecosystems differ from those at lower latitudes, however, in that there is no consistent relationship between CO2 flux and water vapor flux, because vascular plants account for most CO2 flux, whereas mosses account for most water vapor flux[107]. This contrasts with other major biomes on Earth, where these two fluxes are strongly correlated[108].
Within tundra, vegetation strongly influences the winter energy budget through its effects on snow depth and density. Shrubs increase snow depth by reducing the velocity of blowing snow and reducing sublimation rates; models suggest that in northern Alaska this shrub-induced reduction in sublimation can increase ecosystem-scale winter snow accumulation by 20%[109]. By acting as a "snow fence", shrubs also cause snow to accumulate within shrub patches and to be depleted from shrub-free zones, increasing the spatial heterogeneity of snow depth. Snow within shrub canopies is deeper and less dense, which reduces heat transfer through the snowpack and increases winter soil temperatures by 2 °C relative to adjacent shrub-free tundra. Warmer soiltemperatures beneath shrubs may increase winter decomposition and enhance nutrient availability, creating a positive feedback that promotes shrub growth[110].
Midsummer vegetation feedbacks to regional climate are determined largely by midsummer patterns of water and energy exchange[111]. Midsummer albedo is greatest in sedge communities, whose standing dead leaves reflect much of the incoming radiation[112]. Evergreen forests and forest tundra, in contrast, have a particularly low albedo because of the dark absorptive nature of evergreen leaves and the effectiveness of complex forest canopies in capturing light[113]. Tundra and forest tundra canopies dominated by deciduous plants are intermediate in albedo and therefore in the quantity of energy that they absorb and transfer to the atmosphere[114]. A larger proportion of the energy transfer to the atmosphere occurs as sensible heat flux in forests, forest tundra, and shrub tundra than in wet tundra[115].
All arctic ecosystems exhibit greater ground heat flux during summer (5 to 15% of net radiation) than do temperate ecosystems (generally close to zero), due to the strong thermal gradient between the ground surface and permafrost and the long hours of solar radiation[116]. Ground heat fluxes are reduced in tundra ecosystems with a large leaf area, which shades the ground surface[117], or where the ground cover is highly insulative, as with Sphagnum mosses[118]. Grazing and other processes causing surface disturbance increase ground heat flux and thaw depth[119]. Future changes in vegetation driven by climate change are very likely to profoundly alter regional climate.
Summary
Arctic ecosystems tend to accumulate organic matter and elements despite low inputs because organic matter decomposition is very slow. As a result, soil-available elements like N and P place key limits on increases in C fixation and further biomass and organic matter accumulation. Key issues for projecting whole-system responses to climate change include the importance of carbon–nutrient interactions; the interactions of C and nutrient cycles with temperature, water, and snow cover; the magnitude of dissolved organic and inorganic C losses in soil water; and the magnitude and role of wintertime processes. Most disturbances are expected to increase C and element turnover, particularly in soils. This is likely to lead to initial losses of elements but eventual, slow recovery. Individual species and species diversity have clear impacts on element inputs and retention in arctic ecosystems, but their magnitude relative to climate and resource supply is still uncertain. Similarly, the current information about the long-term effects of increasing CO2 concentrations and UV-B radiation levels on whole ecosystems indicates that direct effects of these variables will probably be small relative to changes in soil resources and element turnover. Indirect effects of increasing CO2 concentrations and UV-B radiation levels are likely to be more important at the ecosystem level (e.g., through changes in species composition).
The most important trace gases in arctic ecosystems are CO2 and CH4. Trace-gas exchange with the atmosphere occurs through a set of coupled soil ecosystem processes. Wet and moist tundra environments are known to be significant contributors to atmospheric CH4. However, CH4 is also consumed in aerobic parts of the soil. Methane emissions from the ecosystems are a balance between production and consumption, with production more responsive to warming than consumption. Soil warming in the absence of any other changes is very likely to accelerate emissions. Winter processes and vegetation type also affect CH4 emissions. Nitrous oxide emissions are also sensitive to winter conditions and potential winter warming.
Arctic ecosystems exhibit the largest seasonal changes in energy exchange of any terrestrial ecosystem because of the large changes in albedo from late winter, when snow reflects most incoming radiation, to summer when the ecosystem absorbs most incoming radiation. Vegetation profoundly influences the water and energy exchange of arctic ecosystems. Vascular plants account for most CO2 flux, whereas mosses account for most water vapor flux. Albedo during the period of snow cover declines from tundra to forest tundra to deciduous forest to evergreen forest. Shrubs and trees increase snow depth, which in turn increases ground heat fluxes; ecosystems with a large leaf area and insulating moss carpets reduce ground heat fluxes and conserve permafrost. Future changes in vegetation driven by climate change are very likely to profoundly alter regional climate.
Chapter 7: Arctic Tundra and Polar Desert Ecosystems
7.1 Introduction (Effects of changes in climate and UV radiation levels on function of arctic ecosystems in the short and long term)
7.2 Late-Quaternary changes in arctic terrestrial ecosystems, climate, and ultraviolet radiation levels
7.3 Species responses to changes in climate and ultraviolet-B radiation in the Arctic
7.3.1 Implications of current species distributions for future biotic change
7.3.2 General characteristics of arctic species and their adaptations in the context of changes in climate and ultraviolet-B radiation levels
7.3.3 Phenotypic responses of arctic species to changes in climate and ultraviolet-B radiation
7.3.4 Genetic responses of arctic species to changes in climate and ultraviolet-B radiation levels
7.3.5 Recent and projected changes in arctic species distributions and potential ranges
7.4 Effects of changes in climate and UV radiation levels on structure and function of arctic ecosystems in the short and long term
7.4.1 Ecosystem structure
7.4.2 Ecosystem function
7.5 Effects of climate change on landscape and regional processes and feedbacks to the climate system
7.6 Synthesis: Scenarios of projected changes in the four ACIA regions for 2020, 2050, and 2080
7.7 Uncertainties and recommendations
References
a The biological definition of NEP results in positive values when carbon accumulates in the biosphere, whereas measurements of carbon fluxes and the concept of carbon sinks and sources use a convention of negative fluxes when carbon accumulates in the biosphere.
Citation
Committee, I. (2012). Effects of changes in climate and UV radiation levels on function of arctic ecosystems in the short and long term. Retrieved from http://editors.eol.org/eoearth/wiki/Effects_of_changes_in_climate_and_UV_radiation_levels_on_function_of_arctic_ecosystems_in_the_short_and_long_term- ↑ Jonasson, S., F.S. Chapin III and G.R. Shaver, 2001. Biogeochemistry in the Arctic: patterns, processes and controls. In: E.-D. Schulze, M. Heimann, S.P. Harrison, E.A. Holland, J.J. Lloyd, I.C. Prentice and D. Schimel (eds.). Global Biogeochemical Cycles in the Climate System, pp. 139–150. Academic Press.
- ↑ Michaelson, G.J., C.-L. Ping and J.M. Kimble, 1996. Carbon storage and distribution in tundra soils in Arctic Alaska, U.S.A. Arctic and Alpine Research, 28:414–424.
- ↑ Bliss, L.C., O.W. Heal and J.J. Moore (eds.), 1981. Tundra Ecosystems: A Comparative Analysis. Cambridge University Press, 813pp.
- ↑ Jonasson, S., A. Michelsen and I.K. Schmidt, 1999a. Coupling of nutrient cycling and carbon dynamics in the Arctic, integration of soil microbial and plant processes. Applied Soil Ecology, 11:135–146.
- ↑ Cheng, W. and R.A. Virginia, 1993. Measurement of microbial biomass in arctic tundra soils using fumigation-extraction and substrate-induced respiration procedures. Soil Biology and Biochemistry, 25:135–141.– Hobbie, S.E. and F.S. Chapin III, 1996. Winter regulation of tundra litter carbon and nitrogen dynamics. Biogeochemistry, 35:327–338.– Jonasson, S., A. Michelsen, I.K. Schmidt, E.V. Nielsen and T.V. Callaghan, 1996. Microbial biomass C, N, and P in two arctic soils and responses to addition of NPK fertilizer and sugar: Implications for plant nutrient uptake. Oecologia, 106:507–515.– Jonasson, S., A. Michelsen and I.K. Schmidt, 1999a. Coupling of nutrient cycling and carbon dynamics in the Arctic, integration of soil microbial and plant processes. Applied Soil Ecology, 11:135–146.– Schmidt, I.K., S. Jonasson, G.R. Shaver, A. Michelsen and A. Nordin, 2002. Mineralization and distribution of nutrients in plants and microbes in four arctic ecosystems: responses to warming. Plant and Soil, 242:93–106.
- ↑ Nadelhoffer, W.C. Oechel and E.B. Rastetter, 1992. Global change and the carbon balance of arctic ecosystems. Bioscience, 42:433–441.
- ↑ Kaye, J.P. and S.C. Hart, 1997. Competition for nitrogen between plants and soil microorganisms.Trends in Ecology and Evolution, 12:139–143.
- ↑ Giblin, A.E., K.J. Nadelhoffer, G.R. Shaver, J.A. Laundre and A.J. McKerrow, 1991. Biogeochemical diversity along a riverside toposequence in arctic Alaska. Ecological Monographs, 61:415–435.– Jonasson, S., F.S. Chapin III and G.R. Shaver, 2001. Biogeochemistry in the Arctic: patterns, processes and controls. In: E.-D. Schulze, M. Heimann, S.P. Harrison, E.A. Holland, J.J. Lloyd, I.C. Prentice and D. Schimel (eds.). Global Biogeochemical Cycles in the Climate System, pp. 139–150. Academic Press.
- ↑ Jonasson, S., F.S. Chapin III and G.R. Shaver, 2001. Biogeochemistry in the Arctic: patterns, processes and controls. In: E.-D. Schulze, M. Heimann, S.P. Harrison, E.A. Holland, J.J. Lloyd, I.C. Prentice and D. Schimel (eds.). Global Biogeochemical Cycles in the Climate System, pp. 139–150. Academic Press.
- ↑ McGuire, A.D., J.M. Melillo, D.W. Kicklighter,Y. Pan, X. Xiao, J. Helfrich, B.M. Moore III, C.J. Vorosmarty and A.L. Schloss, 1997. Equilibrium responses of global net primary production and carbon storage to doubled atmospheric carbon dioxide: sensitivity to changes in vegetation nitrogen concentration. Global Biogeochemical Cycles, 11:173–189.
- ↑ Billings,W.D., 1973. Arctic and Alpine vegetations: similarities, differences, and susceptibility to disturbance. Bioscience, 23:697–704.– Jonasson, S., F.S. Chapin III and G.R. Shaver, 2001. Biogeochemistry in the Arctic: patterns, processes and controls. In: E.-D. Schulze, M. Heimann, S.P. Harrison, E.A. Holland, J.J. Lloyd, I.C. Prentice and D. Schimel (eds.). Global Biogeochemical Cycles in the Climate System, pp. 139–150. Academic Press.– Shaver, G.R., J.A. Laundre, A.E. Giblin and K.J. Nadelhoffer, 1996. Changes in live plant biomass, primary production, and species composition along a riverside toposequence in arctic Alaska, U.S.A. Arctic and Alpine Research, 28:363–379.
- ↑ Jonasson, S., F.S. Chapin III and G.R. Shaver, 2001. Biogeochemistry in the Arctic: patterns, processes and controls. In: E.-D. Schulze, M. Heimann, S.P. Harrison, E.A. Holland, J.J. Lloyd, I.C. Prentice and D. Schimel (eds.). Global Biogeochemical Cycles in the Climate System, pp. 139–150. Academic Press.
- ↑ Bliss, L.C. and N.V. Matveyeva, 1992. Circumpolar Arctic vegetation. In: F.S. Chapin III, R.L. Jefferies, J.F. Reynolds, G.R. Shaver, and J. Svoboda (eds.). Arctic Ecosystems in a Changing Climate: An Ecophysiological Perspective, pp. 59–89. Academic Press.
- ↑ Oechel, W.C. and W.D. Billings, 1992. Effects of global change on the carbon balance of Arctic plants and ecosystems. In: F.S. Chapin III, R.L. Jefferies, J.F. Reynolds, G.R. Shaver and J. Svoboda (eds.). Arctic Ecosystems in a Changing Climate: An Ecophysiological Perspective, pp. 139–168. Academic Press.
- ↑ Walker, D.A., N.A. Auerbach, J.G. Bockheim, F.S. Chapin III, W. Eugster, J.Y. King, J.P. McFadden, G.J. Michaelson, F.E. Nelson, W.C. Oechel, C.-L. Ping,W.S. Reeburgh, S. Regli, N.I. Shiklomanov and G.L. Vourlitis, 1998. Energy and trace-gas fluxes across a soil pH boundary in the Arctic. Nature, 394:469–472.– Walker, D.A., W.A. Gould, H.A. Maier and M.K. Raynolds, 2002. The Circumpolar Arctic Vegetation Map: AVHRR-derived base maps, environmental controls, and integrated mapping procedures. International Journal of Remote Sensing, 23:4551–4570.– Walker, M.D., D.A. Walker and K.R. Everett, 1989. Wetland Soils and Vegetation, Arctic Foothills, Alaska. U.S. Fish and Wildlife Service Biological Report 89(7), 89pp.– Walker, M.D., D.A. Walker and N.A.Auerbach, 1994. Plant communities of a tussock tundra landscape in the Brooks Range Foothills, Alaska. Journal of Vegetation Science, 5:843–866.
- ↑ Shaver, G.R., W.D. Billings, F.S. Chapin III, A.E. Giblin, K.J. Nadelhoffer, W.C. Oechel and E.B. Rastetter, 1992. Global change and the carbon balance of arctic ecosystems. Bioscience, 42:433–441.
- ↑ Gold, W.G. and L.C. Bliss, 1995.The nature of water limitations for plants in a high arctic polar desert. In: T.V. Callaghan, W.C. Oechel, T. Gilmanov, J.I. Holten, B. Maxwell, U. Molau, B. Sveinbjornsson and M.J. Tyson (eds.). Global Change and Arctic Terrestrial Ecosystems, pp. 149–155. Ecosystems Research Report 10. European Commission, Brussels.
- ↑ Giblin, A.E., K.J. Nadelhoffer, G.R. Shaver, J.A. Laundre and A.J. McKerrow, 1991. Biogeochemical diversity along a riverside toposequence in arctic Alaska. Ecological Monographs, 61:415–435.
- ↑ Hershey, A.E., W.B. Bowden, L.A. Deegan, J.E. Hobbie, B.J. Peterson, G.W. Kipphut, G.W. Kling, M.A. Lock, R.W. Merritt, M.C. Miller, J.R. Vestal and J.A. Schuldt, 1997. The Kuparuk River: A long-term study of biological and chemical processes in an arctic river. In: A.M. Milner and M.W. Oswood (eds.). Freshwaters of Alaska: Ecological Syntheses. Ecological Studies 119:107–130. Springer-Verlag.– Kling, G.W., G.W. Kipphut, M.C. Miller and W.J. O'Brien, 2000. Integration of lakes and streams in a landscape perspective: the importance of material processing on spatial patterns and temporal coherence. Freshwater Biology, 43:477–497.– Oechel, W.C., G.L. Vourlitis, J. Verfaillie Jr., T. Crawford, S. Brooks, E. Dumas, A. Hope, D. Stow, B. Boynton, V. Nosov and R. Zulueta, 2000a. A scaling approach for quantifying the net CO2 flux of the Kuparuk River Basin, Alaska. Global Change Biology, 6(S1):160–173.– Steiglitz, M., A. Giblin, J. Hobbie, M. Williams and G. Kling, 2000. Simulating the effects of climate change and climate variability on carbon dynamics in Arctic tundra. Global Biogeochemical Cycles, 14:1123–1136.
- ↑ Oechel, W.C., S.J. Hastings, G.L. Vourlitis, M. Jenkins, G. Riechers and N. Grulke, 1993. Recent change of arctic tundra ecosystems from a net carbon dioxide sink to a source. Nature, 361:520–523.– Oechel, W.C., G.L. Vourlitis, S.J. Hastings, R.C. Zulueta, L. Hinzman and D. Kane, 2000b. Acclimation of ecosystem CO2 exchange in the Alaskan Arctic in response to decadal climate warming. Nature, 406:978–981.
- ↑ Nordström, C., H. Soegaard, T.R. Christensen,T. Friborg and B.U. Hansen, 2001. Seasonal carbon dioxide balance and respiration of a high-arctic fen ecosystem in NE-Greenland.Theoretical and Applied Climatology, 70(1–4):149–166.– Oechel, W.C., G.L. Vourlitis, J. Verfaillie Jr., T. Crawford, S. Brooks, E. Dumas, A. Hope, D. Stow, B. Boynton, V. Nosov and R. Zulueta, 2000a. A scaling approach for quantifying the net CO2 flux of the Kuparuk River Basin, Alaska. Global Change Biology, 6(S1):160–173.– Søgaard, H., C. Nordstrøm, T. Friborg, B.U. Hansen, T.R. Christensen and C. Bay, 2000. Trace gas exchange in a high-arctic valley. 3: Integrating and scaling CO2 fluxes from canopy to landscape using flux data, footprint modeling, and remote sensing. Global Biogeochemical Cycles, 14:725–744.– Vourlitis, G.L. and W.C. Oechel, 1997. Landscape-scale CO2, H2O vapor, and energy flux of moist-wet coastal tundra ecosystems over two growing seasons. Journal of Ecology, 85:575–590.– Vourlitis, G.L. and W.C. Oechel, 1999. Eddy covariance measurements of CO2 and energy fluxes of an Alaskan tussock tundra ecosystem. Ecology, 80:686–701.
- ↑ Oechel, W.C., G.L. Vourlitis, J. Verfaillie Jr., T. Crawford, S. Brooks, E. Dumas, A. Hope, D. Stow, B. Boynton, V. Nosov and R. Zulueta, 2000a. A scaling approach for quantifying the net CO2 flux of the Kuparuk River Basin, Alaska. Global Change Biology, 6(S1):160–173.
- ↑ Clein, J.S., B.L. Kwiatkowski, A.D. McGuire, J.E. Hobbie, E.B. Rastetter, J.M. Melillo and D.W. Kicklighter, 2000. Modelling carbon responses of tundra ecosystems to historical and projected climate: A comparison of a plot- and a global-scale ecosystem model to identify process-based uncertainties. Global Change Biology, 6(S1):127–140.– McGuire, A.D., J.S. Clein, J.M. Melillo, D.W. Kicklighter, R.A. Meier, C.J. Vorosmarty and M.C. Serreze, 2000. Modeling carbon responses of tundra ecosystems to historical and projected climate: sensitivity of pan-arctic carbon storage to temporal and spatial variation in climate. Global Change Biology, 6(S1):141–159.– McKane, R.B., E.B. Rastetter, G.R. Shaver, K.J. Nadelhoffer, A.E. Giblin, J.A. Laundre and F.S. Chapin III, 1997. Climatic effects on tundra carbon storage inferred from experimental data and a model. Ecology, 78:1170–1187.
- ↑ Shaver, G.R. and F.S. Chapin III, 1991. Production: biomass relationships and element cycling in contrasting Arctic vegetation types. Ecological Monographs, 61:1–31.– Shaver, G.R., L.C. Johnson, D.H. Cades, G. Murray, J.A. Laundre, E.B. Rastetter, K.J. Nadelhoffer and A.E. Giblin, 1998. Biomass and CO2 flux in wet sedge tundras: responses to nutrients, temperature, and light. Ecological Monographs, 68:75–97.
- ↑ Callaghan, T.V. and S. Jonasson, 1995. Arctic terrestrial ecosystems and environmental change. Philosophical Transactions of the Royal Society of London A, 352:259–276.
- ↑ Chapin, F.S. III, L. Moilanen and K. Kielland, 1993. Preferential use of organic nitrogen for growth by a non-mycorrhizal Arctic sedge. Nature, 361:150–153.– Hobbie, S.E., 1995. Direct and indirect species effects on biogeochemical processes in arctic ecosystems. In: F.S. Chapin III and C. Körner (eds.). Arctic and Alpine Biodiversity: Patterns, Causes, and Ecosystem Consequences. Ecological Studies, 113:213–224. Springer-Verlag.
- ↑ Shaver, G.R., M.S. Bret-Harte, M.H. Jones, J. Johnstone, L. Gough, J. Laundre and F.S. Chapin III, 2001. Species composition interacts with fertilizer to control long-term change in tundra productivity. Ecology, 82:3163–3181.
- ↑ Bret-Harte, M.S., G.R. Shaver, J.P. Zoerner, J.F. Johnstone, J.L.Wagner, A.S. Chavez, R.F. Gunkelman, S.C. Lippert and J.A. Laundre, 2001. Developmental plasticity allows Betula nana to dominate tundra subjected to an altered environment. Ecology, 82:18–32.
- ↑ Jónsdóttir, I.S., T.V. Callaghan and J.A. Lee, 1995. Fate of added nitrogen in a moss-sedge Arctic community and effects of increased nitrogen deposition. Science of the Total Environment, 161:677–685.
- ↑ Sturm, M., C. Racine and K.Tape, 2001b. Climate change: Increasing shrub abundance in the Arctic. Nature, 411:546–547.
- ↑ Shaver, G.R., W.D. Billings, F.S. Chapin III, A.E. Giblin, K.J. Nadelhoffer,W.C. Oechel and E.B. Rastetter, 1992. Global change and the carbon balance of arctic ecosystems. Bioscience, 42:433–441.
- ↑ Chapin, F.S. III, P.C. Miller, W.D. Billings and P.I. Coyne, 1980. Carbon and nutrient budgets and their control in coastal tundra. In: J. Brown, P.C. Miller, L.L. Tieszen, and F.L. Bunnell (eds.). An Arctic Ecosystem:The Coastal Tundra at Barrow, Alaska, pp. 458–482. Dowden, Hutchinson and Ross, Pennsylvania.
- ↑ Hobbie, S.E., 1996. Temperature and plant species control over litter decomposition in Alaskan tundra. Ecological Monographs, 66:503–522.
- ↑ Hobbie, S.E., J.P. Schimel, S.E. Trumbore and J.R. Randerson, 2000. Controls over carbon storage and turnover in high-latitude soils. Global Change Biology, 6(S1):196–210.– Williams, M.,W. Eugster, E.B. Rastetter, J.P. McFadden and F.S. Chapin III, 2000. The controls on net ecosystem productivity along an Arctic transect: a model comparison with flux measurements. Global Change Biology, 6(S1):116–126.
- ↑ Chapin, F.S. III, P.C. Miller, W.D. Billings and P.I. Coyne, 1980. Carbon and nutrient budgets and their control in coastal tundra. In: J. Brown, P.C. Miller, L.L. Tieszen, and F.L. Bunnell (eds.). An Arctic Ecosystem: The Coastal Tundra at Barrow, Alaska, pp. 458–482. Dowden, Hutchinson and Ross, Pennsylvania.
- ↑ Nadelhoffer, K.J., A.E. Giblin, G.R. Shaver and J.A. Laundre, 1991. Effects of temperature and substrate quality on element mineralization in six Arctic soils. Ecology, 72:242–253.– Peterson, B.J., T.L. Corliss, K. Kriet and J.E. Hobbie, 1992. Nitrogen and phosphorus concentrations and export for the Upper Kuparuk River on the North Slope of Alaska in 1980. Hydrobiologia, 240:61–69.– Shaver, G.R., W.D. Billings, F.S. Chapin III, A.E. Giblin, K.J. Nadelhoffer, W.C. Oechel and E.B. Rastetter, 1992. Global change and the carbon balance of arctic ecosystems. Bioscience, 42:433–441.
- ↑ Gold, W.G. and L.C. Bliss, 1995.The nature of water limitations for plants in a high arctic polar desert. In: T.V. Callaghan, W.C. Oechel, T. Gilmanov, J.I. Holten, B. Maxwell, U. Molau, B. Sveinbjornsson and M.J. Tyson (eds.). Global Change and Arctic Terrestrial Ecosystems, pp. 149–155. Ecosystems Research Report 10. European Commission, Brussels.
- ↑ Williams, M., E.B. Rastetter, G.R. Shaver, J.E. Hobbie, E. Carpino and B.L. Kwiatkowski, 2001. Primary production of an arctic watershed: an uncertainty analysis. Ecological Applications, 11:1800–1816.
- ↑ Williams, M., E.B. Rastetter, G.R. Shaver, J.E. Hobbie, E. Carpino and B.L. Kwiatkowski, 2001. Primary production of an arctic watershed: an uncertainty analysis. Ecological Applications, 11:1800–1816.
- ↑ Williams, M. and E.B. Rastetter, 1999. Vegetation characteristics and primary productivity along an arctic transect: implications for scaling up. Journal of Ecology, 87:885–898.
- ↑ Jonasson, S., F.S. Chapin III and G.R. Shaver, 2001. Biogeochemistry in the Arctic: patterns, processes and controls. In: E.-D. Schulze, M. Heimann, S.P. Harrison, E.A. Holland, J.J. Lloyd, I.C. Prentice and D. Schimel (eds.). Global Biogeochemical Cycles in the Climate System, pp. 139–150. Academic Press.– Shaver, G.R., W.D. Billings, F.S. Chapin III, A.E. Giblin, K.J. Nadelhoffer, W.C. Oechel and E.B. Rastetter, 1992. Global change and the carbon balance of arctic ecosystems. Bioscience, 42:433–441.
- ↑ Chapin, F.S. III and G.R. Shaver, 1985b. Arctic. In: B.F. Chabot and H.A. Mooney (eds.). Physiological Ecology of North American Plant Communities, pp. 16–40. Chapman and Hall.
- ↑ Williams, M.,W. Eugster, E.B. Rastetter, J.P. McFadden and F.S. Chapin III, 2000. The controls on net ecosystem productivity along an Arctic transect: a model comparison with flux measurements. Global Change Biology, 6(S1):116–126.– Williams, M., E.B. Rastetter, G.R. Shaver, J.E. Hobbie, E. Carpino and B.L. Kwiatkowski, 2001. Primary production of an arctic watershed: an uncertainty analysis. Ecological Applications, 11:1800–1816.
- ↑ Kling, G.W., G.W. Kipphut and M.C. Miller. 1991. Arctic lakes and streams as gas conduits to the atmosphere: Implications for tundra carbon budgets. Science, 251:298–301.– Kling, G.W., G.W. Kipphut and M.C. Miller, 1992. The flux of CO2 and CH4 from lakes and rivers in arctic Alaska. Hydrobiologia, 240:23–36.
- ↑ Coyne, P.I. and J.J. Kelley, 1971. Release of carbon dioxide from frozen soil to the Arctic atmosphere. Nature, 234:407–408.
- ↑ Fahnestock, J.T., M.H. Jones and J.M. Welker, 1999. Wintertime CO2 efflux from Arctic soils: Implications for annual carbon budgets. Global Biogeochemical Cycles, 13:775–780.– Hobbie, S.E., J.P. Schimel, S.E. Trumbore and J.R. Randerson, 2000. Controls over carbon storage and turnover in high-latitude soils. Global Change Biology, 6(S1):196–210.– Oechel, W.C., G. Vourlitis and S.J. Hastings, 1997. Cold season CO2 emission from Arctic soils. Global Biogeochemical Cycles, 11:163–172.– Welker, J.M., J.T. Fahnestock and M.H. Jones, 2000. Annual CO2 flux in dry and moist Arctic tundra: field responses to increases in summer temperatures and winter snow depth. Climatic Change, 44:139–150.
- ↑ Grogan, P., L. Illeris, A. Michelsen and S. Jonasson, 2001. Respiration of recently-fixed plant carbon dominates mid-winter ecosystem CO2 production in sub-arctic heath tundra. Climatic Change, 50:129–142.
- ↑ Shaver, G.R., W.D. Billings, F.S. Chapin III, A.E. Giblin, K.J. Nadelhoffer, W.C. Oechel and E.B. Rastetter, 1992. Global change and the carbon balance of arctic ecosystems. Bioscience, 42:433–441.
- ↑ Gold, W.G. and L.C. Bliss, 1995.The nature of water limitations for plants in a high arctic polar desert. In: T.V. Callaghan, W.C. Oechel, T. Gilmanov, J.I. Holten, B. Maxwell, U. Molau, B. Sveinbjornsson and M.J. Tyson (eds.). Global Change and Arctic Terrestrial Ecosystems, pp. 149–155. Ecosystems Research Report 10. European Commission, Brussels.
- ↑ Chapin, F.S. III, P.C. Miller, W.D. Billings and P.I. Coyne, 1980. Carbon and nutrient budgets and their control in coastal tundra. In: J. Brown, P.C. Miller, L.L. Tieszen, and F.L. Bunnell (eds.). An Arctic Ecosystem: The Coastal Tundra at Barrow, Alaska, pp. 458–482. Dowden, Hutchinson and Ross, Pennsylvania.
- ↑ Back, J., S. Neuvonen and S. Huttunen, 1994. Pine needle growth and fine structure after prolonged acid rain treatment in the subarctic. Plant, Cell and Environment, 17:1009–1021.
- ↑ Chapin, D.M. and C.S. Bledsoe, 1992. Nitrogen fixation in arctic plant communities. In: F.S. Chapin III, R.L. Jefferies, J.F. Reynolds, G.R. Shaver and J. Svoboda (eds.). Arctic Ecosystems in a Changing Climate: An Ecophysiological Perspective, pp. 301–319. Academic Press.– Lennihan, R., D.M. Chapin and L.G. Dickson, 1994. Nitrogen fixation and photosynthesis in high arctic forms of Nostoc commune. Canadian Journal of Botany, 72:940–945.
- ↑ Christensen, T.R., A. Michelsen and S. Jonasson, 1999a. Exchange of CH4 and N2O in a subarctic heath soil: effects of inorganic N and P and amino acid addition. Soil Biology and Biochemistry, 31:637–641.
- ↑ Hershey, A.E., W.B. Bowden, L.A. Deegan, J.E. Hobbie, B.J. Peterson, G.W. Kipphut, G.W. Kling, M.A. Lock, R.W. Merritt, M.C. Miller, J.R. Vestal and J.A. Schuldt, 1997. The Kuparuk River: A long-term study of biological and chemical processes in an arctic river. In: A.M. Milner and M.W. Oswood (eds.). Freshwaters of Alaska: Ecological Syntheses. Ecological Studies 119:107–130. Springer-Verlag.
- ↑ Dormann, C.F. and S.J. Woodin, 2002. Climate change in the Arctic: using plant functional types in a meta-analysis of field experiments. Functional Ecology, 16:4–17.
- ↑ Dormann, C.F. and S.J. Woodin, 2002. Climate change in the Arctic: using plant functional types in a meta-analysis of field experiments. Functional Ecology, 16:4–17.– Shaver, G.R. and S. Jonasson, 2000. Response of Arctic ecosystems to climate change: results of long-term field experiments in Sweden and Alaska. Polar Research, 18:245–252.
- ↑ Oechel, W.C., G.L. Vourlitis, S.J. Hastings, R.P. Ault Jr. and P. Bryant, 1998.The effects of water table manipulation and elevated temperature on the net CO2 flux of wet sedge tundra ecosystems. Global Change Biology, 4:77–90.– Oechel, W.C., G.L. Vourlitis, J. Verfaillie Jr., T. Crawford, S. Brooks, E. Dumas, A. Hope, D. Stow, B. Boynton, V. Nosov and R. Zulueta, 2000a. A scaling approach for quantifying the net CO2 flux of the Kuparuk River Basin, Alaska. Global Change Biology, 6(S1):160–173.– Shaver, G.R., L.C. Johnson, D.H. Cades, G. Murray, J.A. Laundre, E.B. Rastetter, K.J. Nadelhoffer and A.E. Giblin, 1998. Biomass and CO2 flux in wet sedge tundras: responses to nutrients, temperature, and light. Ecological Monographs, 68:75–97.
- ↑ Grulke, N., G.H. Riechers, W.C. Oechel, U. Hjelm and C. Jaeger, 1990. Carbon balance in tussock tundra under ambient and elevated atmospheric CO2. Oecologia, 83:485–494.– Hartley, A.E., C. Neill, J.M. Melillo, R. Crabtree and F.P. Bowles, 1999. Plant performance and soil nitrogen mineralization in response to simulated climate change in subarctic dwarf shrub heath. Oikos, 86:331–343.– Oechel, W.C., S. Cowles, N. Grulke, S.J. Hastings, B. Lawrence, T. Prudhomme, G. Riechers, B. Strain, D. Tissue and G. Vourlitis, 1994. Transient nature of CO2 fertilization in Arctic tundra. Nature, 371:500–503.
- ↑ Schmidt, I.K., S. Jonasson and A. Michelsen, 1999. Mineralization and microbial immobilization of N and P in arctic soils in relation to season, temperature and nutrient amendment. Applied Soil Ecology, 11:147–160.
- ↑ Luo, Y., S. Wan, D. Hui and L.L. Wallace, 2001. Acclimatization of soil respiration to warming in a tall grass prairie. Nature, 413:622–625.– Hartley, A.E., C. Neill, J.M. Melillo, R. Crabtree and F.P. Bowles, 1999. Plant performance and soil nitrogen mineralization in response to simulated climate change in subarctic dwarf shrub heath. Oikos, 86:331–343.
- ↑ Shaver, G.R. and F.S. Chapin III, 1991. Production: biomass relationships and element cycling in contrasting Arctic vegetation types. Ecological Monographs, 61:1–31.
- ↑ Callaghan, T.V. and S. Jonasson, 1995. Arctic terrestrial ecosystems and environmental change. Philosophical Transactions of the Royal Society of London A, 352:259–276.
- ↑ Myneni, R.B., C.D. Keeling, C.J. Tucker, G. Asrar and R.R. Nemani, 1997. Increased plant growth in the northern high latitudes from 1981–1991. Nature, 386:698–702.
- ↑ Christensen, T.R., S. Jonasson, T.V. Callaghan and M. Havström, 1999b. On the potential CO2 releases from tundra soils in a changing climate. Applied Soil Ecology, 11:127–134.– Grogan, P., L. Illeris, A. Michelsen and S. Jonasson, 2001. Respiration of recently-fixed plant carbon dominates mid-winter ecosystem CO2 production in sub-arctic heath tundra. Climatic Change, 50:129–142.
- ↑ Niemi, R., P.J. Martikainen, J. Silvola, A. Wulff, S. Turtola and T. Holopainen, 2002. Elevated UV-B radiation alters fluxes of methane and carbon dioxide in peatland microcosms. Global Change Biology, 8:361–371.
- ↑ Gehrke, C., U. Johanson, T.V. Callaghan, D. Chadwick and C.H. Robinson, 1995. The impact of enhanced ultraviolet-B radiation on litter quality and decomposition processes in Vaccinium leaves from the Subarctic. Oikos, 72:213–222.– Searles, P.S., S.D. Flint, S.B. Diaz, M.C. Rousseaux, C.L. Ballaré and M.M. Caldwell, 1999. Solar ultraviolet-B radiation influence on Sphagnum bog and Carex fen ecosystems: first field season findings in Tierra del Fuego, Argentina. Global Change Biology, 5:225–234.– Sonesson, M., B.A. Carlsson,T.V. Callaghan, S. Halling, L.O. Björn, M. Bertgren and U. Johanson, 2002. Growth of two peat-forming mosses in subarctic mires: species interactions and effects of simulated climate change. Oikos, 99:151–160.
- ↑ Bret-Harte, M.S., G.R. Shaver, J.P. Zoerner, J.F. Johnstone, J.L. Wagner, A.S. Chavez, R.F. Gunkelman, S.C. Lippert and J.A. Laundre, 2001. Developmental plasticity allows Betula nana to dominate tundra subjected to an altered environment. Ecology, 82:18–32.
- ↑ Hobbie, S.E. and F.S. Chapin III, 1998.The response of tundra plant biomass, aboveground production, nitrogen, and CO2 flux to experimental warming. Ecology, 79:1526–1544.– Quested, H.M., J.H.C. Cornelissen, M.C. Press, T.V. Callaghan, R. Aerts, F. Trosien, P. Riemann, D. Gwynn-Jones, A. Kondratchuk and S.E. Jonasson, 2003. Decomposition of sub-arctic plants with differing nitrogen economies:A functional role for hemiparasites. Ecology, 84:3209–3221.
- ↑ Bret-Harte, M.S., G.R. Shaver and F.S. Chapin III, 2002. Primary and secondary stem growth in arctic shrubs: implications for community response to environmental change. Journal of Ecology, 90:251–267.– Quested, H.M., J.H.C. Cornelissen, M.C. Press, T.V. Callaghan, R. Aerts, F.Trosien, P. Riemann, D. Gwynn-Jones, A. Kondratchuk and S.E. Jonasson, 2003. Decomposition of sub-arctic plants with differing nitrogen economies:A functional role for hemiparasites. Ecology, 84:3209–3221.– Shaver, G.R., J. Canadell, F.S. Chapin III, J. Gurevitch, J. Harte, G. Henry, P. Ineson, S. Jonasson, J. Melillo, L. Pitelka and L. Rustad, 2000. Global warming and terrestrial ecosystems: A conceptual framework for analysis. Bioscience, 50:871–882.
- ↑ McFadden, J.P., G.E. Liston, M. Sturm, R.A. Pielke Sr. and F.S. Chapin III, 2001. Interactions of shrubs and snow in arctic tundra: Measurements and models. In: A.J. Dolman, A.J. Hall, M.L. Kavvas,T. Oki and J.W. Pomeroy (eds.). Soil-Vegetation-Atmosphere Transfer Schemes and Large-Scale Hydrological Models. IAHS Publication 270:317–325. International Association of Hydrological Sciences, UK.– Sturm, M., J.P. McFadden, G.E. Liston, F.S. Chapin III, C.H. Racine and J. Holmgren, 2001a. Snow-shrub interactions in Arctic tundra: A hypothesis with climatic implications. Journal of Climate, 14:336–344.
- ↑ Joabsson, A. and T.R. Christensen, 2001. Methane emissions from wetlands and their relationship with vascular plants: an Arctic example. Global Change Biology, 7:919–932.– Niemi, R., P.J. Martikainen, J. Silvola, A. Wulff, S. Turtola and T. Holopainen, 2002. Elevated UV-B radiation alters fluxes of methane and carbon dioxide in peatland microcosms. Global Change Biology, 8:361–371.– Öquist, M.G. and B.H. Svensson, 2002. Vascular plants as regulators of methane emissions from a subarctic mire ecosystem. Journal of Geophysical Research, 107(D21):4580.
- ↑ Joabsson, A. and T.R. Christensen, 2001. Methane emissions from wetlands and their relationship with vascular plants: an Arctic example. Global Change Biology, 7:919–932.
- ↑ Gough, L., G.R. Shaver, J. Carroll, D.L. Royer and J.A. Laundre, 2000. Vascular plant species richness in Alaskan arctic tundra: the importance of soil pH. Journal of Ecology, 88:54–66.
- ↑ Michelsen, A., I.K. Schmidt, S. Jonasson, C. Quarmby and D. Sleep, 1996. Leaf 15N abundance of subarctic plants provides field evidence that ericoid, ectomycorrhizal and non- and arbuscular mycorrhizal species access different sources of soil nitrogen. Oecologia, 105:53–63.– Michelsen, A., C. Quarmby, D. Sleep and S. Jonasson, 1998. Vascular plant 15N abundance in heath and forest tundra ecosystems is closely correlated with presence and type of mycorrhizal fungi in roots. Oecologia, 115:406–418.– Nadelhoffer, K.J., G. Shaver, B. Fry, A.E. Giblin, L. Johnson and R. McKane, 1996. 15N natural abundances and N use by tundra plants. Oecologia, 107:386–394.
- ↑ McKane, R.B., L.C. Johnson, G.R. Shaver, K.J. Nadelhoffer, E.B. Rastetter, B. Fry, A.E. Giblin, K. Kielland, B.L. Kwiatkowski, J.A. Laundre and G. Murray, 2002. Resource-based niches provide a basis for plant species diversity and dominance in arctic tundra. Nature, 415:68–71.
- ↑ Fetcher, N., 1985. Effects of removal of neighboring species on growth, nutrients, and microclimate of Eriophorum vaginatum. Arctic and Alpine Research, 17:7–17.– Jonasson, S., 1992. Plant responses to fertilization and species removal in tundra related to community structure and clonality. Oikos, 63:420–429.– Shevtsova, A., A. Ojala, S. Neuvonen, M. Vieno and E. Haukioja, 1995. Growth and reproduction of dwarf shrubs in a subarctic plant community: annual variation and above-ground interactions with neighbours. Journal of Ecology, 83:263–275.– Shevtsova, A., E. Haukioja and A. Ojala, 1997. Growth response of subarctic dwarf shrubs, Empetrum nigrum and Vaccinium vitis-ideae, to manipulated environmental conditions and species removal. Oikos, 78:440–458.
- ↑ Bubier, J.L. and T.R. Moore, 1994. An ecological perspective on methane emissions from northern wetlands. Trends in Ecology and Evolution, 9:460–464.– Joabsson, A. and T.R. Christensen, 2001. Methane emissions from wetlands and their relationship with vascular plants: an Arctic example. Global Change Biology, 7:919–932.– Joabsson, A., T.R. Christensen and B. Wallén, 1999. Vascular plant controls on methane emissions from northern peatforming wetlands. Trends in Ecology and Evolution, 14:385–388.– Niemi, R., P.J. Martikainen, J. Silvola, A. Wulff, S. Turtola and T. Holopainen, 2002. Elevated UV-B radiation alters fluxes of methane and carbon dioxide in peatland microcosms. Global Change Biology, 8:361–371.
- ↑ Joabsson, A. and T.R. Christensen, 2001. Methane emissions from wetlands and their relationship with vascular plants: an Arctic example. Global Change Biology, 7:919–932.– Ström, L., A. Ekberg and T.R. Christensen, 2003. The effect of vascular plants on carbon turnover and methane emissions from a tundra wetland. Global Change Biology, 9:1185–1192.
- ↑ Niemi, R., P.J. Martikainen, J. Silvola, A. Wulff, S. Turtola and T. Holopainen, 2002. Elevated UV-B radiation alters fluxes of methane and carbon dioxide in peatland microcosms. Global Change Biology, 8:361–371.
- ↑ Panikov, N.S., 1999. Fluxes of CO2 and CH4 in high latitude wetlands: measuring, modelling and predicting response to climate change. Polar Research, 18(2):237–244.
- ↑ Christensen, T.R., N. Panikov, M. Mastepanov, A. Joabsson, A. Stewart, M. Oquist, M. Sommerkorn, S. Reynaud and B. Svensson, 2003a. Biotic controls on CO2 and CH4 exchange in wetlands - a closed environment study. Biogeochemistry, 64:337–354.
- ↑ Bartlett, K.B. and R.C. Harriss, 1993. Review and assessment of methane emissions from wetlands. Chemosphere, 26:261–320.– Fung, I., J. John, J. Lerner, E. Matthews, M. Prather, L.P. Steele and P.J. Fraser, 1991.Three-dimensional model synthesis of the global methane cycle. Journal of Geophysical Research, 96:13033–13065.
- ↑ Joabsson, A. and T.R. Christensen, 2001. Methane emissions from wetlands and their relationship with vascular plants: an Arctic example. Global Change Biology, 7:919–932.– Schimel, J.P., 1995. Plant transport and methane production as controls on methane flux from arctic wet meadow tundra. Biogeochemistry, 28:183–200.– Ström, L., A. Ekberg and T.R. Christensen, 2003. The effect of vascular plants on carbon turnover and methane emissions from a tundra wetland. Global Change Biology, 9:1185–1192.– Whalen, S.C. and W.S. Reeburgh, 1992. Interannual variations in tundra methane emission: a 4-year time series at fixed sites. Global Biogeochemical Cycles, 6:139–159.
- ↑ Christensen,T.R., A. Michelsen and S. Jonasson, 1999a. Exchange of CH4 and N4O in a subarctic heath soil: effects of inorganic N and P and amino acid addition. Soil Biology and Biochemistry, 31:637–641.– Panikov, N.S., 1999. Fluxes of CO2 and CH4 in high latitude wetlands: measuring, modelling and predicting response to climate change. Polar Research, 18(2):237–244.– Sjögersten, S. and P.A. Wookey, 2002. Spatio-temporal variability and environmental controls of methane fluxes at the forest-tundra ecotone in the Fennoscandian mountains. Global Change Biology, 8:885–895.– Whalen, S.C. and W.S. Reeburgh, 1992. Interannual variations in tundra methane emission: a 4-year time series at fixed sites. Global Biogeochemical Cycles, 6:139–159.
- ↑ Nordström, C., H. Soegaard, T.R. Christensen, T. Friborg and B.U. Hansen, 2001. Seasonal carbon dioxide balance and respiration of a high-arctic fen ecosystem in NE-Greenland. Theoretical and Applied Climatology, 70(1–4):149–166.– Oechel, W.C., G.L. Vourlitis, S.J. Hastings, R.C. Zulueta, L. Hinzman and D. Kane, 2000b. Acclimation of ecosystem CO2 exchange in the Alaskan Arctic in response to decadal climate warming. Nature, 406:978–981.– Søgaard, H., C. Nordstrøm, T. Friborg, B.U. Hansen, T.R. Christensen and C. Bay, 2000. Trace gas exchange in a high-arctic valley. 3: Integrating and scaling CO2 fluxes from canopy to landscape using flux data, footprint modeling, and remote sensing. Global Biogeochemical Cycles, 14:725–744.
- ↑ Christensen, T.R., T. Friborg, M. Sommerkorn, J. Kaplan, L. Illeris, H. Søgaard, C. Nordstrøm and S. Jonasson, 2000. Trace gas exchange in a high-arctic valley. 1:Variations in CO2 and CH4 flux between tundra vegetation types. Global Biogeochemical Cycles, 14:701–714.– Friborg,T., T.R. Christensen, B.U. Hansen, C. Nordstrøm and H. Søgaard, 2000. Trace gas exchange in a high arctic valley 2: Landscape CH4 fluxes measured and modeled using eddy correlation data. Global Biogeochemical Cycles, 14:715–724.– Whalen, S.C. and W.S. Reeburgh, 1990. A methane flux transect along the Trans-Alaska Pipeline Haul Road. Tellus B: 42:237–249.
- ↑ Christensen, T.R., A. Michelsen and S. Jonasson, 1999a. Exchange of CH4 and N2O in a subarctic heath soil: effects of inorganic N and P and amino acid addition. Soil Biology and Biochemistry, 31:637–641.
- ↑ Conrad, R., 1996. Soil microorganisms as controllers of atmospheric trace gases (H2, CO, CH4, OCS, N2O, and NO). Microbiological Reviews, 60(4):609–640.– Whalen, S.C. and W.S. Reeburgh, 2001. Carbon monoxide consumption in upland boreal forest soils. Soil Biology and Biogeochemistry, 33:1329–1338.
- ↑ Conrad, R., 1996. Soil microorganisms as controllers of atmospheric trace gases (H2, CO, CH4, OCS, N2O, and NO). Microbiological Reviews, 60(4):609–640.
- ↑ Guenther, A., P. Zimmerman and M. Wildermuth, 1993. Natural volatile organic compound emission rate estimates for U.S. woodland landscapes. Atmospheric Environment, 28:1197–1210.– Isidorov, V.A. and M. Jdanova, 2002.Volatile organic compounds from leaves litter. Chemosphere, 48(9):975–979.– Isidorov, V.A, I.G. Zenkevich and B.V. Ioffe, 1983. Methods and results of gas chromatographic-mass spectrometric determination of volatile organic substances in an urban atmosphere. Atmospheric Environment, 17(7):1347–1353.
- ↑ Dimmer, C.H., P.G. Simmonds, G. Nickless and M.R. Bassford, 2001. Biogenic fluxes of halomethanes from Irish peatland ecosystems. Atmospheric Environment, 35:321–330.
- ↑ Legrand, M., 1995. Atmospheric chemistry changes versus past climate inferred from polar ice cores. In: R.J. Charlson and J. Heintzenberg (eds.). Aerosol Forcing of Climate, pp. 123–151,Wiley.
- ↑ Conrad, R., 1996. Soil microorganisms as controllers of atmospheric trace gases (H2, CO, CH4, OCS, N2O, and NO). Microbiological Reviews, 60(4):609–640.
- ↑ Conrad, R., 1996. Soil microorganisms as controllers of atmospheric trace gases (H2, CO, CH4, OCS, N2O, and NO). Microbiological Reviews, 60(4):609–640.
- ↑ Harriss, R., K. Bartlett, S. Frolking and P. Crill, 1993. Methane emissions from northern high-latitude wetlands. In: R.S. Oremland (ed.). Biogeochemistry of Global Change: Radiatively Active Trace Gases, pp. 449–486. Chapman & Hall.– Roulet, N.T., T. Moore, J. Bubier and P. Lafleur, 1992. Northern fens: methane flux and climatic change. Tellus B, 44:100–105.
- ↑ Christensen, T.R. and P. Cox, 1995. Response of methane emission from Arctic tundra to climatic change: results from a model simulation. Tellus B:47:301–309.
- ↑ Panikov, N.S. and S.N. Dedysh, 2000. Cold season CH4 and CO2 emission from boreal peat bogs (West Siberia): Winter fluxes and thaw activation dynamics. Global Biogeochemical Cycles, 14(4):1071–1080.
- ↑ Crill, P., K.B. Bartlett and N.T. Roulet, 1992. Methane flux from boreal peatlands. Suo, 43:173–182.– Harriss, R., K. Bartlett, S. Frolking and P. Crill, 1993. Methane emissions from northern high-latitude wetlands. In: R.S. Oremland (ed.). Biogeochemistry of Global Change: Radiatively Active Trace Gases, pp. 449–486. Chapman & Hall.
- ↑ Christensen, T.R., A. Ekberg, L. Ström, M. Mastepanov, N. Panikov, M. Öquist, B.H. Svensson, H. Nykänen, P.J. Martikainen and H. Oskarsson, 2003b. Factors controlling large scale variations in methane emissions from wetlands. Geophysical Research Letters, 30(7):1414, doi:10.1029/2002GL016848.– Ström, L., A. Ekberg and T.R. Christensen, 2003. The effect of vascular plants on carbon turnover and methane emissions from a tundra wetland. Global Change Biology, 9:1185–1192.
- ↑ Davidson, E.A. and J.P. Schimel, 1995. Microbial processes of production and consumption of nitric oxide, nitrous oxide and methane. In: P.A. Matson and R.C. Harriss (eds.). Biogenic Trace Gases: Measuring Emissions from Soil and Water, pp. 327–357. Blackwell Science.
- ↑ Christensen, T.R., A. Michelsen and S. Jonasson, 1999a. Exchange of CH4 and N2O in a subarctic heath soil: effects of inorganic N and P and amino acid addition. Soil Biology and Biochemistry, 31:637–641.
- ↑ Dorland, S. and E.G. Beauchamp, 1991. Denitrification and ammonification at low soil temperatures. Canadian Journal of Soil Science, 71:293–303.– Malhi, S.S., W.B. McGill and M. Nyborg, 1990. Nitrate losses in soils: effect of temperature, moisture and substrate concentration. Soil Biology and Biochemistry, 22:733–737.
- ↑ Christensen, S. and B.T. Christensen, 1991. Organic matter available for denitrification in different soil fractions: Effect of freeze/thaw cycles and straw disposal. Journal of Soil Science, 42:637–647.
- ↑ Papen, H. and K. Butterbach-Bahl, 1999. A 3-year continuous record of nitrogen trace gas fluxes from untreated and limed soil of a N-saturated spruce and beech forest ecosystem in Germany. Part 1: N2O emissions. Journal of Geophysical Research, 104(D15):18487–18503.
- ↑ Christensen, T.R., A. Michelsen and S. Jonasson, 1999a. Exchange of CH4 and N2O in a subarctic heath soil: effects of inorganic N and P and amino acid addition. Soil Biology and Biochemistry, 31:637–641.
- ↑ Eugster, W., W.R. Rouse, R.A. Pielke, J.P. McFadden, D.D. Baldocchi, T.G.F. Kittel, F.S. Chapin III, G.E. Liston, P.L. Vidale, E. Vaganov and S. Chambers, 2000. Land-atmosphere energy exchange in Arctic tundra and boreal forest: available data and feedbacks to climate. Global Change Biology, 6(S1):84–115.
- ↑ McFadden, J.P., W. Eugster and F.S. Chapin III, 2003. A regional study of the controls on water vapor and CO2 exchange in Arctic tundra. Ecology, 84:2762–2776.
- ↑ Kelliher, F.M., R. Leuning, M.R. Raupach and E.-D. Schulze, 1995. Maximum conductances for evaporation from global vegetation types. Agricultural and Forest Meteorology, 73:1–16.– Schulze, E.-D., F.M. Kelliher, C. Körner, J. Lloyd and R. Leuning, 1994. Relationships among maximum stomatal conductance, ecosystem surface conductance, carbon assimilation rate, and plant nitrogen nutrition: a global ecology scaling exercise. Annual Review of Ecology and Systematics, 25:629–662.
- ↑ Sturm, M., J.P. McFadden, G.E. Liston, F.S. Chapin III, C.H. Racine and J. Holmgren, 2001a. Snow-shrub interactions in Arctic tundra: A hypothesis with climatic implications. Journal of Climate, 14:336–344.
- ↑ Sturm, M., J.P. McFadden, G.E. Liston, F.S. Chapin III, C.H. Racine and J. Holmgren, 2001a. Snow-shrub interactions in Arctic tundra: A hypothesis with climatic implications. Journal of Climate, 14:336–344.
- ↑ Chapin, F.S. III, W. Eugster, J.P. McFadden, A.H. Lynch and D.A. Walker, 2000a. Summer differences among Arctic ecosystems in regional climate forcing. Journal of Climate, 13:2002–2010.
- ↑ Chapin, F.S. III, W. Eugster, J.P. McFadden, A.H. Lynch and D.A.Walker, 2000a. Summer differences among Arctic ecosystems in regional climate forcing. Journal of Climate, 13:2002–2010.– McFadden, J.P., F.S. Chapin III and D.Y. Hollinger, 1998. Subgrid-scale variability in the surface energy balance of Arctic tundra. Journal of Geophysical Research, 103(D22):28947–28961.
- ↑ Chapin, F.S. III, A.D. McGuire, J. Randerson, R. Pielke Sr., D. Baldocchi, S.E. Hobbie, N. Roulet, W. Eugster, E. Kasischke, E.B. Rastetter, S.A. Zimov and S.W. Running, 2000b. Arctic and boreal ecosystems of western North America as components of the climate system. Global Change Biology, 6(S1):211–223.– Eugster, W., W.R. Rouse, R.A. Pielke, J.P. McFadden, D.D. Baldocchi, T.G.F. Kittel, F.S. Chapin III, G.E. Liston, P.L. Vidale, E. Vaganov and S. Chambers, 2000. Land-atmosphere energy exchange in Arctic tundra and boreal forest: available data and feedbacks to climate. Global Change Biology, 6(S1):84–115.
- ↑ Baldocchi, D., F.M. Kelliher, T.A. Black and P.G. Jarvis, 2000. Climate and vegetation controls on boreal zone energy exchange. Global Change Biology, 6(S1):69–83.– Chapin, F.S. III, A.D. McGuire, J. Randerson, R. Pielke Sr., D. Baldocchi, S.E. Hobbie, N. Roulet, W. Eugster, E. Kasischke, E.B. Rastetter, S.A. Zimov and S.W. Running, 2000b. Arctic and boreal ecosystems of western North America as components of the climate system. Global Change Biology, 6(S1):211–223.
- ↑ Boudreau, L.D. and W.R. Rouse, 1995. The role of individual terrain units in the water balance of wetland tundra. Climate Research, 5:31–47.– Chapin, F.S. III, W. Eugster, J.P. McFadden, A.H. Lynch and D.A.Walker, 2000a. Summer differences among Arctic ecosystems in regional climate forcing. Journal of Climate, 13:2002–2010.– Eugster, W., W.R. Rouse, R.A. Pielke, J.P. McFadden, D.D. Baldocchi, T.G.F. Kittel, F.S. Chapin III, G.E. Liston, P.L. Vidale, E. Vaganov and S. Chambers, 2000. Land-atmosphere energy exchange in Arctic tundra and boreal forest: available data and feedbacks to climate. Global Change Biology, 6(S1):84–115.– Lafleur, P.M., W.R. Rouse and D.W. Carlson, 1992. Energy balance differences and hydrologic impacts across the northern treeline. International Journal of Climatology, 12:193–203.– McFadden, J.P., F.S. Chapin III and D.Y. Hollinger, 1998. Subgrid-scale variability in the surface energy balance of Arctic tundra. Journal of Geophysical Research, 103(D22):28947–28961.
- ↑ Chapin, F.S. III, W. Eugster, J.P. McFadden, A.H. Lynch and D.A.Walker, 2000a. Summer differences among Arctic ecosystems in regional climate forcing. Journal of Climate, 13:2002–2010.
- ↑ McFadden, J.P., F.S. Chapin III and D.Y. Hollinger, 1998. Subgrid-scale variability in the surface energy balance of Arctic tundra. Journal of Geophysical Research, 103(D22):28947–28961.
- ↑ Beringer, J., A.H. Lynch, F.S. Chapin III, M. Mack and G.B. Bonan, 2001a. The representation of Arctic soils in the Land Surface Model: the importance of mosses. Journal of Climate, 14:3324–3335.
- ↑ Walker, D.A., N.A. Auerbach, J.G. Bockheim, F.S. Chapin III, W. Eugster, J.Y. King, J.P. McFadden, G.J. Michaelson, F.E. Nelson, W.C. Oechel, C.-L. Ping, W.S. Reeburgh, S. Regli, N.I. Shiklomanov and G.L.Vourlitis, 1998. Energy and trace-gas fluxes across a soil pH boundary in the Arctic. Nature, 394:469–472.