Climate change and forest distribution in the Arctic
This is Section 14.11 of the Arctic Climate Impact Assessment.
Lead Author: Glenn P. Juday; Contributing Authors: Valerie Barber, Paul Duffy, Hans Linderholm, Scott Rupp, Steve Sparrow, Eugene Vaganov, John Yarie; Consulting Authors: Edward Berg, Rosanne D’Arrigo, Olafur Eggertsson,V.V. Furyaev, Edward H. Hogg, Satu Huttunen, Gordon Jacoby, V.Ya. Kaplunov, Seppo Kellomaki, A.V. Kirdyanov, Carol E. Lewis, Sune Linder, M.M. Naurzbaev, F.I. Pleshikov, Ulf T. Runesson,Yu.V. Savva, O.V. Sidorova,V.D. Stakanov, N.M.Tchebakova, E.N.Valendik, E.F.Vedrova, Martin Wilmking.
Contents
Historic examples of treeline movement (14.11.1)
The contrast between the realm of life where trees dominate the environment and the very different realm where much shorter herbaceous and low woody vegetation is the exclusive plant cover is one of the most visually striking landscape characteristics of the circumpolar Arctic. A number of questions about arctic and associated alpine treelines have attracted scientific interest for well over a century. Treeline questions have been studied from a number of different perspectives, but nearly all are at least implicitly connected with the question of how cold temperatures limit tree growth and survival. Because of this overriding interest in temperature limits, and because of the ubiquitous occurrence of treelines across the Arctic and the high state of preservation of dead trees in the cold environment of the Arctic, treeline studies have much to offer on the specific question of climate warming and cooling. This article focuses on some recent studies that shed light on the long-term history of dynamics and movement of tree limits as related to specific temperature-controlled processes. These studies provide a perspective of long-term continuity, and insight into the mechanisms that recent temperature increases have affected, and that warming projected by the Arctic Climate Impact Assessment (ACIA)-designated models could affect.
Northern Eurasia (14.11.1.1)
Between 9,000 and 7,000 years before present (Early Holocene), forest occupied what is now treeless tundra nearly extending to the arctic coastline throughout northern Russia[1]. These results are based on earlier subfossil wood collections carried out by Russian scientists as well as modern collections of subfossil wood from the Kola, Yamal, and Taymir Peninsulas, and at the mouth of the Lena River. Subsequently, the greatest retreat of forest and expansion of tundra (compared to the modern position of the tree-line) took place between 4,000 and 3,000 years BP. During the period of maximum forest advance, the mean July temperature in northern Russia (at the coast) was 2.5 to 7.0 °C higher than the present-day mean, based on modern tree growth relationships to temperature. The northward advance of the tree-line reflected a series of other environmental changes in the Arctic, including increased solar insolation, reduced sea-ice cover in the Arctic Ocean, increased climate continentality, and a significant intrusion of warm North Atlantic water into the Arctic[2]. This documented record of past forest advance suggests that there is a solid basis for projecting similar tree-line change from scenarios that project similar temperature increases. It also suggests that the components of ecosystems present today have the capacity to respond and adjust to such climate fluctuations.
A reconstruction of tree-line movement in the Swedish Scandes Mountains using 173 dated remains of pine wood reveals that there has been a gradual decrease in the elevation of the tree-line (with small fluctuations), generally consistent with climate cooling. Elevational decline in tree-line as the result of decreasing temperatures began sometime after 10,700 calendar years BP. Although the rate of tree-line recession was greater before about 8,000 years BP than after, generally the tree-line evidence here indicates a smooth long-term temperature decrease with only a few minor, brief warming and cooling episodes. The total elevational retreat of tree-line was estimated to be 500 meters (m), corresponding to a temperature decrease of 6 to 8 °C. Since the beginning of the 20th century, however, a local warming of about 1 °C has been associated with an upward movement of more than 100 m, which represents the largest adjustment of tree-line position in the last 3,000 years[3].
Yamal Peninsula (14.11.1.2)
Detailed analyses of the dynamics of polar treeline on the Yamal Peninsula are presented in Hantemirov[4], Hantemirov and Shiyatov[5], and Shiyatov et al.[6]. Holocene wood deposits in the southern Yamal Peninsula include a large quantity of subfossil tree remains, including stems, roots, and branches. This is the result of intensive accumulation and conservation of buried wood in permafrost. The existence of extensive frozen subfossil wood in the southern Yamal Peninsula in what is now the southern tundra zone was first noted and described by B.M. Zhitkov in 1912 during an ornithological expedition through the area. Tikhomirov[7] showed that tree remains conserved in peat sediments were direct evidence that the northern treeline in the warmest period of the Holocene reached the central regions of the Yamal Peninsula (up to 70° N). Today the polar treeline is considerably further south on the Peninsula (67°30’ N).
Systematic collection of subfossil wood samples started in 1982 in the watersheds of the Khadyta, the Yadayakhodiyakha, and the Tanlova Rivers in the southern Yamal Peninsula, located between 67°00’ and 67°50’ N and 68°30’ and 71°00’ E. River flow in this area is from north to south, excluding the possibility that wood was transported to the collection site from more southerly locations; thus, there is very high confidence that the region experienced a considerably warmer summer climate in the relatively recent past.
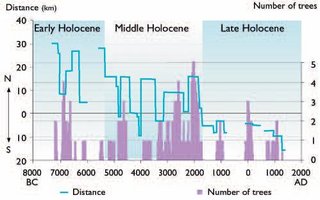
Radiocarbon dating of the subfossil wood (53 dates) was cross dated with ring series from the samples, allowing the construction of a continuous tree-ring chronology 7,314 years in length. Absolute dates can generally be assigned to the recovered wood remains. The result makes it possible to reconstruct the dynamics of tree limits on the Yamal Peninsula during the Holocene (Fig. 14.27). From at least 10,000 to 9,000 years BP, trees grew across most of the Peninsula. The most favorable conditions for tree growth (almost certainly warm summers) occurred from 7200 to 6000 BC. From about 6000 to 5600 BC, climatic conditions became less favorable for tree growth (almost certainly cooler summers), but trees persisted and did not retreat to the south. Beginning about 5400 BC, trees were displaced southward. The forest stand density also greatly decreased during this period, and it can be considered as a transition to the next stage of the Holocene. From 5400 until 1700 BC, the polar treeline was still located at 69° N, well north of the present-day position. In unfavorable periods (4500–3900 BC and 3600–3400 BC), tree survival was mainly restricted to the river corridors, but in climatically more favorable periods (5200–4500 BC, 3900–3600 BC, and 3400–1800 BC), forests grew on hills and raised surfaces beyond the rivers.
One of the most important periods of displacement of polar treeline to the south and major reduction in the density and productivity of forest stands occurred about 1700 BC. This stage can be regarded as the end of the Middle Holocene and the beginning of the modern stage of treeline evolution on the Yamal Peninsula. During the last 1,700 years, forest–tundra and forest associations have been primarily restricted to river valleys in the southern part of the Peninsula. Somewhat more favorable conditions occurred from 1200 to 900 BC, from 100 BC to AD 200 and during the Medieval Warming Period (MWP) (AD 700–1400).
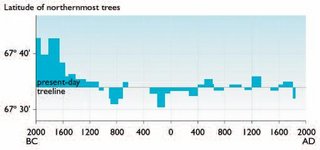
Treeline dynamics for the last 4,000 years of the Holocene were reconstructed with even greater precision using more than 500 cross-dated tree stems with known coordinates of their burial places in valleys of different [[river]s] on the southern-Yamal Peninsula (Fig. 14.28). Treeline displacements northward and southward were relatively small and less important during the last 3,600 years than those that occurred in the previous few millennia. Treeline generally moved at the most 5 km to the south of the present-day treeline, and subsequently northward only to the present boundary of open woodland in the river valleys. However, one particularly noteworthy major displacement of treeline to the south occurred during the second half of the 17th century BC. In this relatively short period (not exceeding 100 years), the boundary of larch open woodland moved southward nearly 15 to 20 kilometers (km), and the treeline retreated a further 8 to 10 km during the next 700 years. This major displacement of treeline in the 17th century BC appears to have been driven by strongly inclement climatic conditions (cold summers), representing the lowest reconstructed summer temperatures in the entire series. It was in the years immediately after 1657 BC that the temperature decreased sharply. Fourteen years during the interval 1630 to 1611 BC appear to have been extremely cold, reaching a nadir in two specific years, 1626 and 1625 BC. No other period during the reconstruction is even close. Moreover, it is clear that in 1625 BC, a severe freeze occurred in the middle of the summer (as indicated by characteristic anatomical structures of freeze injury in the tree rings). It is very probable that this short-term extreme climate event represented climate cooling following one of the largest volcanic eruptions of the last few millennia, which happened in about 1628 BC (possibly the eruption of the Santorini volcano in the eastern Mediterranean). The cooling appears to have reinforced another closely spaced cooling event that preceded it. The earlier of the two periods of extreme cold temperatures began sometime after 1657 BC, but in this case, it is difficult to determine the cause[10]. These events were the final circumstances that resulted in the most significant southward retreat of treeline during at least the last 4,000 years. In spite of extremely favorable summer warmth that returned afterward and even persisted at various intervals, the treeline never returned to its previous boundary.
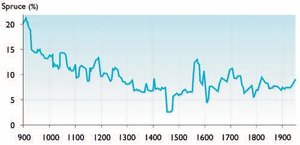
Another important result of dendrochronological dating of large samples of subfossil wood is the ability to calculate the relative abundance of Siberian spruce in forest stands of the area, which is an index or proxy for the degree of continentality of the climate. Figure 3 shows the change in the proportion of spruce in forest stands (the remaining part is all larch). In the first six centuries, from AD 900 to 1500, the proportion of spruce decreased from 22% to 3–5%. After that, the percentage of spruce stabilized in the range of 7-10%. The 20th century is characterized by an increasing percentage of spruce in forest stands in the valley of the River Khadytayakha, and a weak northward advance of the polar treeline.
Polar Ural Mountains (14.11.1.3)
Significant spatial and temporal changes took place in the upper treeline ecotone in the Polar Ural Mountains (66°–67° N, 65°–66° E) during the last millennium[12]. Within the treeline ecotone, located between 100 and 350 m above sea level, open forests of Siberian larch dominate. Patches of closed forests of mixed larch and Siberian spruce grow at lower altitudes in the ecotone. Up to the present, these forests have largely developed under conditions of very little human influence. A large number of wood remnants persist on the ground at elevations up to 60 to 80 m above the present treeline, and within the ecotone between forest and tundra. These wood remains have been preserved for up to 1,300 years because of the low rate of wood decomposition in the severe cold. The wood remnants provide material to extend a ring-width chronology back to AD 645, and to date the lifespan of a large quantity of dead trees.
In order to estimate the downward displacement of the altitudinal treeline (the highest altitudinal position of open forest over the last millennium), a transect 430 m long and 20 m wide was set up on the southeastern slope of Rai-lz Massif, from the highest location of larch wood remnants (340 m above sea level) down to the present upper treeline (280 m). The transect was divided into 10 x 10 m quadrants. All of the wood remnants were mapped and wood sections cut from the base of the trunk and roots were collected from each individual tree. The calendar year of establishment and death for each tree was determined by cross-dating. Altogether, the life history of 270 dead trees was defined. In addition, 16 young living trees and seedlings were also mapped and their age determined.
Using these data, stages in the overall downward displacement of the upper treeline over the last 1,150 years were reconstructed (Table 14.8). This time interval was divided into 5 periods, distinguished by differing directions of treeline shifts (rise or retreat in elevation) and differing rates of displacement. During the earliest period (430 years, AD 850–1280), the upper treeline rose from 305 to 340 m above sea level, an average of 1 to 2 m per decade. The highest altitudinal position reached by the treeline and the densest and most productive larch stands of the last millennium occurred in the 13th century. The second period (300 years, AD 1280–1580) was characterized by a substantial retreat of the upper treeline (from 340 m down to 295 m) at a mean rate of 2 to 3 m per decade. During the third period (210 years, AD 1580–1790), the treeline retreat stopped. The calculated rise (from 295 to 305 m, 0.5–1.0 m/decade) was not significant. The most extreme retreat was seen in the fourth period (120 years, AD 1790–1910), during which the upper treeline receded from 305 m to 270 m, an average of 2-4 m per decade. During this fourth period, the upper treeline was at its lowest altitudinal position of the millennium. In the last period, from 1910 to the present, vigorous forest establishment took place on sites that were forested during the Middle Ages. The rate of change was the highest of the millennium (from 270 to 308 m, 4–6 m/decade).
Table 14.8. Altitudinal displacement of the upper treeline in the Polar Ural Mountains during the last 1150 years[13]. | |||
Period (AD) |
Number of years |
Change in treeline | |
Direction |
Rate (m/decade) | ||
850 –1280 |
430 |
rise |
1–2 |
1280 –1580 |
300 |
retreat |
2–3 |
1580 –1790 |
210 |
rise |
0.5–1.0 |
1790 –1910 |
120 |
retreat |
2–4 |
1910 –2000 |
90 |
rise |
4–6 |
The last period of expansion of forest vegetation is being studied using both direct and indirect evidence (old terrestrial, aerial, and satellite photographs; repeated stand descriptions of permanent plots and transects; morphology and age structure of stands; large-scale mapping within the ecotone; and meteorological and dendroclimatic data). Table 14.9 shows the change in area of different types of forest–tundra ecosystems within the ecotone during the 20th century. These data were obtained at the time of large-scale (1:10,000) mapping of the key study area (3,085 ha) located at the bottom of Tchernaya Mountain. Three maps were produced that show the spatial distribution of these types of forest–tundra ecosystems in the beginning, middle, and end of the 20th century. Change in area was estimated for two periods: the 50 years from 1910 to 1960, and the 40 years from 1960 to 2000.
Table 14.9. Area change in different types of forest–tundra ecosystems from 1910 to 2000 in the Polar Ural Mountains[14]. | ||||||||
Tundra with individual trees |
Sparse growth of trees |
Open forest |
Closed forest | |||||
Area (ha) |
Change (ha/decade) |
Area (ha) |
Change (ha/decade) |
Area (ha) |
Change (ha/decade) |
Area (ha) |
Change (ha/decade) | |
1910 |
2403 |
349 |
328 |
5 |
||||
-76 |
37 |
24 |
15 | |||||
1960 |
2021 |
535 |
450 |
79 |
||||
-26 |
-52 |
36 |
42 | |||||
2000 |
1917 |
327 |
593 |
258 |
Between 1910 and 2000, the area of tundra with only scattered individual trees decreased significantly from 2,403 to 1,917 ha, or from 78 to 62% of the main study area. The greatest rate of change (-76 ha/decade) was observed during the first 50 years, when isolated seedling establishment above the treeline was the most intensive. Data obtained on the change in area of the sparse trees mapping unit is very interesting because during the first period the change was positive (+37 ha/decade) but during the second period it was negative (-52 ha/ decade). The decrease occurred when young established trees began producing seeds, resulting in an increase in stand density (many stands changed from tundra with isolated trees into open forest). This led to the great increase in open-forest area between 1960 and 2000 (from 450 to 593 ha, +36 ha/decade). However, the most impressive changes were seen in the case of closed forests. The area of closed forest increased from 5 to 258 ha over 90 years with the transformation of open forests into closed forests. To date, more than 550 repeated terrestrial photographs have been taken which can be used to reconstruct stand parameters for the middle of the 20th century.
Temperature increases during the 20th century were the major cause of the expansion of trees in the treeline ecotone. The mean June temperature at the Salekhard weather station (50 km east of the study area) increased from 7.2 °C (1883–1919) to 8.5 °C (1920–1998). The July mean increased from 13.8 to 14.3 °C. Mean temperatures in the winter months (November–March) increased from -20.8 to -19.6 °C. The average June–July temperature, which is critical for tree growth, increased from 10.5 to 11.4 °C (0.9 °C). This means that the June–July isotherm rose 120 to 130 m in altitude (in this area the elevational temperature gradient is 0.7 °C/ 100 m). However, the mean rise of the upper treeline was only 20-40 m owing to a deficiency of viable seeds on sites remote from fertile trees and stands.
In this area, larch cones open and seeds are disseminated only on days with elevated temperatures, typically from the end of June to the end of July[15]. Wind is the primary method of seed dissemination; other means of dispersal, such as animals and birds, are insignificant. Because seed dissemination takes place in summer when snow cover is absent, it is difficult for seeds to be transported upwards over long distances. Heavy larch seeds are carried no more then 40 to 60 m from the tree and most of these seeds become lodged in lichen–moss and shrub layers that prevent them from germinating successfully. That is why abundant seedling establishment took place only close to individual trees and within existing stands.
Impressive changes have occurred in the structure of existing stands during the last 90 years. Most sites with trees have become much denser and more productive (up to 4-5 times) and many tundra sites located within the treeline ecotone have been afforested. The degree of afforestation increased from 22 to 38% (based on data from Table 14.9). Thus, many factors affecting the forest–tundra ecotone position reacted to summer temperature changes. However, for the purpose of climatic reconstruction, the best proxies for reconstructing summer temperature are those obtained from existing stands (tree rings, biomass, stand and canopy density, degree of afforestation) rather than treeline movement, which experiences lag effects. Although the displacement rate of the upper treeline is a good reflection of long-term climatic fluctuations (Table 14.8), reconstruction of actual temperature changes is complicated because of the response lag caused by the slow growth of seedlings and the lack of seeds on remote sites. For example, the recent warming observed in summer months is of the same degree as inferred for the Middle Ages (about 1 °C) but the upper treeline has not yet reached the altitudinal position where forests grew in the 13th century. To overcome such discrepancies, it is necessary to use corrective factors. These will be different for each period and study area. For example, in the Polar Ural Mountains over the 90 years of study, the upper treeline rose 20 to 40 m in altitude but the June–July temperature isotherm rose 120 to 130 m. Therefore, reconstructed temperatures based on recent treeline movement should be increased by a factor of four.
Recent treeline movement in the Polar Ural Mountains can be confirmed as a widespread phenomenon by comparison of high-resolution satellite images. The satellite and in situ data analysis shows an increasing proportion of the area with higher stand crown closure. Specifically, in 1968 about 23.5% of the area supported stands with crown closure ?30%; in 1998 this area had increased to 50%. The border of stands with closure ?10% moved between 100 and 400 m horizontally, depending on the site. Some areas that were tree-covered before the Little Ice Age still do not support any trees.
Northeast Canada (14.11.1.4)
The Treeline does not always fluctuate in a straightforward way in response to the control of direct climate warming and cooling on the establishment and death of trees from seed, as illustrated in northeastern Canada. Treeline populations in northern Quebec are primarily single-species stands of black spruce, surviving in the upright growth form in protected sites, or in a damaged, low growth form where spruce are exposed to wind and snow abrasion above the snowpack. Spruce macrofossils are generally absent in the northern Quebec tundra zone, and the forest limit and soil charcoal limit occur together, strongly suggesting that the forest limit has remained stable during the last 2,000 to 3,000 years. Apparently, climate change does not easily trigger treeline advance or retreat in this location[16]. However, the character of the forest near the treeline has changed over the last few millennia. The Hustich[17] hypothesis suggests that the regenerative capacity of forests decreases from south to north. At the treeline of northeastern Canada, summer climate is too cold for frequent seed production, although black spruce produce cones that fail to complete only the last stages of development[18]. The black spruce present today were established thousands of years ago in northern Quebec and have persisted by layering since then. Sporadic and patchy fires kill black spruce in this environment and, in the absence of seed production from nearby survivors, trees are eliminated in the local fire patches. By this process, the treeline of today, which is out of equilibrium with the environment that created it, has developed a more irregular and patchy boundary with time[19].
Since the 1960s and 1970s, small-tree stands have emerged above the snowpack, triggered by less harsh conditions associated with snowier winters in the last few decades[20]. This process decreases the numbers of low, damaged growth-form individuals in the population, and increases the numbers of upright, tree-form stems. The temperature increases projected by the ACIA-designated models are likely to be sufficient to provide the final requirement of summer warmth that would result in viable seed production at treeline in northeastern Canada. The initial effect of warming is very likely to induce viable seed production within the forest–tundra zone, resulting in infilling of the patchy forest–tundra border. It is possible that production of viable seeds at the absolute tree limit would begin seed rain onto the tundra, a process that probably has not occurred in appreciable amounts for thousands of years.
Scenarios of future treeline movement (14.11.2)
White et al.[21] developed a global vegetation model to project how increasing atmospheric CO2 concentrations and other parameters, such as air temperature and nitrogen deposition, affect vegetation composition and distribution and carbon sequestration in biomass and [[soil]s] north of 50° N. The model, Hybrid v4.1, is a non-equilibrium, dynamic global vegetation model, with a sub-daily time step, driven by increasing CO2 and transient climate output from the Hadley Centre GCM (HadCM2) with simulated daily and interannual variability. Three emissions scenarios[22] were used: IS92a, which results in an atmospheric CO2 concentration of 790 ppm by 2100; CO2 stabilization at 750 ppm by 2225; and CO2 stabilization at 550 ppm by 2150. Land use and future nitrogen deposition were not included. The model projects an expansion of the area of coniferous boreal forest and mixed/temperate forests of the Northern Hemisphere by about 50%. This forest expansion mainly displaces tundra, driven by the direct effects of rising CO2 levels and temperatures on tree photosynthesis and growth. The forest expansion and an associated increased carbon sink also depend on the indirect effects of increased nitrogen deposition and improved water-use efficiency. However, the model operates with only positive growth responses to increased temperatures, and new findings, such as negative growth responses in treeline trees to increased temperatures (Section 14.7.3.1 (Climate change and forest distribution in the Arctic)) and human activities forcing the treeline southward[23], suggest that the modeled increase in carbon uptake driven by forest expansion is unlikely to be fully realized.
The central Canadian Arctic is a region of low topographic relief and few barriers to tree migration, and is often depicted as an area of major northward tree movement in a straightforward response to increasing temperatures[24]. The BIOME 1.1 model of potential natural vegetation under doubled atmospheric CO2 conditions projects that about 60% of global tundra will be displaced, largely through the expansion of boreal forest[25]. When the BIOME 1.1 model is forced with tripled CO2 concentrations (see Section 4.4.1, Fig. 4.12b (Climate change and forest distribution in the Arctic)), it projects that boreal forest will replace 70% of the tundra of northern Europe and virtually all the European Arctic coast will be occupied by what is today recognized as southern taiga or boreal forest[26]. These vegetation models of boreal forest response to climate change generally produce outputs based on the final adjustment of trees to occupy all climatically suitable areas. However, a variety of processes can reduce the potential for tree expansion or create environments that preclude tree establishment or survival, such as paludification[27]. There is increasing evidence in the Russian Arctic of southward displacement of trees that are subject to strong maritime climate influences or intensified human land uses such as reindeer production systems[28]. At the large scale, future northward movement of the treeline in response to climate change of the magnitude projected by the ACIA-designated models is very likely. However, a considerable lag period is possible, for example because of challenges to tree establishment (e.g., poor seedbed conditions in tundra) that must be overcome by repeated occurrences of low-probability events. Finally, environmental complexity and novelty in an altered, warmer world is likely to produce unexpected vegetation patterns at a local or regional scale.
Chapter 14: Forests, Land Management, and Agriculture
14.1. Introduction (Climate change and forest distribution in the Arctic)
14.2. The boreal forest: importance and relationship to climate
14.3. Land tenure and management in the boreal region
14.4. Use and evaluation of the ACIA scenarios
14.5. Agriculture (Agriculture in the Arctic)
14.6. Tree rings and past climate
14.7. Direct climate effects on tree growth
14.8. Climate change and insects as a forest disturbance
14.9. Climate change and fire
14.10. Climate change in relation to carbon uptake and carbon storage
14.11. Climate change and forest distribution
14.12. Effects of ultraviolet-B on forest vegetation
14.13. Critical research needs
References
Citation
Committee, I. (2012). Climate change and forest distribution in the Arctic. Retrieved from http://editors.eol.org/eoearth/wiki/Climate_change_and_forest_distribution_in_the_Arctic- ↑ MacDonald, G.M., A.A.Velichko, C.V. Kremenetski, O.K. Borisova, A.A. Goleva, A.A. Andreev, L.C. Cwynar, R.T. Riding, S.L. Forman, T.W.D. Edwards, R. Aravena, D. Hammarlund, J.M. Szeicz and V.N. Gattaulin, 2000. Holocene treeline history and climate change across Northern Eurasia. Quaternary Research, 53:302–311.
- ↑ MacDonald, G.M., A.A.Velichko, C.V. Kremenetski, O.K. Borisova, A.A. Goleva, A.A. Andreev, L.C. Cwynar, R.T. Riding, S.L. Forman, T.W.D. Edwards, R. Aravena, D. Hammarlund, J.M. Szeicz and V.N. Gattaulin, 2000. Holocene treeline history and climate change across Northern Eurasia. Quaternary Research, 53:302–311.
- ↑ Kullman, L., 2001. 20th century climate warming and tree-limit rise in the southern Scandes of Sweden. Ambio, 30(2):72–80.
- ↑ Hantemirov, R.M., 1999. Tree-ring reconstruction of summer temperatures on a north of Western Siberia for the last 3248 years. Siberian Ecological Journal, 6(2):185–191.
- ↑ Hantemirov, R.M. and S.G. Shiyatov, 1999. Main stages of woody vegetation development on the Yamal Peninsula in the Holocene. Russian Journal of Ecology, 30(3):141–147.–Hantemirov, R.M. and S.G. Shiyatov, 2002. A continuous multimillennial ring-width chronology in Yamal, northwestern Siberia. The Holocene, 12(6):717–726. Hard, J.S., 1985. Spruce beetles attack slowly growing spruce. Forest Science, 31:839–850.
- ↑ Shiyatov, S.G., R.M. Hantemirov, F.H. Schweingruber, K.R. Briffa and M. Moell, 1996. Potential long-chronology development on the Northwest Siberian Plain: Early results. Dendrochronologia, 14:13–29.
- ↑ Tikhomirov, B. A., 1941. About the forest phase in the post-glacial history of vegetation in a north of Siberia and its relics in a modern tundra. Materials on Flora and Vegetation History of USSR,V.1:315–374.
- ↑ Hantemirov, R.M. and S.G. Shiyatov, 1999. Main stages of woody vegetation development on the Yamal Peninsula in the Holocene. Russian Journal of Ecology, 30(3):141–147.
- ↑ Hantemirov, R.M. and S.G. Shiyatov, 2002. A continuous multimillennial ring-width chronology in Yamal, northwestern Siberia. The Holocene, 12(6):717–726.
- ↑ Grudd, H., K.R. Briffa, B.E. Gunnarson and H. W. Linderholm, 2000. Swedish tree rings provide new evidence in support of a major, widespread environmental disruption in 1628 BC. Geophysical Research Letters, 27:2957–2960.
- ↑ Shiyatov, S.G., 2003. Rates of change in the upper treeline ecotone in the Polar Ural Mountains. PAGES News, 11(1):8–10.
- ↑ Shiyatov, S.G., 1993. The upper timberline dynamics during the last 1100 years in the Polar Ural Mountains. In: B. Frenzel (ed.). Oscillations of the Alpine and Polar Tree Limit in the Holocene, Palaeoclimate Research, 9:195–203.–Shiyatov, S.G., 1995. Reconstruction of climate and the upper timberline dynamics since AD 745 by tree-ring data in the Polar Ural Mountains. In: P. Heikinheimo (ed.). Proceedings of the SILMU International Conference on Past, Present and Future Climate, Helsinki,August 1995. Publications of the Academy of Finland, 6/95:144–147.–Shiyatov, S.G., 2003. Rates of change in the upper treeline ecotone in the Polar Ural Mountains. PAGES News, 11(1):8–10.
- ↑ Shiyatov, S.G., 2003. Rates of change in the upper treeline ecotone in the Polar Ural Mountains. PAGES News, 11(1):8–10.
- ↑ Shiyatov, S.G., 2003. Rates of change in the upper treeline ecotone in the Polar Ural Mountains. PAGES News, 11(1):8–10.
- ↑ Shiyatov, S.G., 1966. The time of dispersion of Siberian larch seeds in north-western part of its areal space and role of that factor in mutual relation between forest and tundra. In: Problems of Physiology and Geobotany. Publication of the Sverdlovsk Branch of the All-Union Botanical Society, 4: 109–113. (In Russian)
- ↑ Lavoie, C. and S. Payette, 1996. The long-term stability of the boreal forest limit in subarctic Québec. Ecology, 77(4):1226–1233.
- ↑ Hustich, I., 1966. On the forest-tundra and the northern tree lines. Annales Universitatis Turkuensis Series A II, 36:7–47.
- ↑ Sirois, L., 1999. The sustainability of development in northern Quebec forests: social opportunities and ecological challenges. In: S. Kankaanpaa, T. Tasanen and M.-L. Sutinen (eds.). Sustainable Development in the Northern Timberline Forests. Proceedings of the Timberline Workshop, May 10–11, 1998,Whitehorse, Canada, pp. 17–27. Finnish Forest Research Institute, Helsinki.
- ↑ Payette, S. and R. Gagnon, 1985. Late Holocene deforestation and tree regeneration in the forest-tundra of Quebec. Nature, 313:570–572.
- ↑ Pereg, D. and S. Payette, 1998. Development of black spruce growth forms at treeline. Plant Ecology, 138:137–147.
- ↑ White, A., M.R.G. Cannell and A.D. Friend, 2000. The high latitude terrestrial carbon sink: a model analysis. Global Change Biology, 6:227–246.
- ↑ IPCC, 1995. Climate Change 1994: Radiative Forcing of Climate Change and an Evaluation of the IPCC 1992 Emission Scenarios. J. T. Houghton, L.G. Meira Filho, J. Bruce, H. Lee, B. A. Callander, E. Haites, N. Harris and K. Maskell (eds.). Cambridge University Press, 339p.
- ↑ Vlassova,T.K., 2002. Human impacts on the tundra-taiga zone dynamics: the case of the Russian lesotundra. Ambio Special Report, 12:30–36.
- ↑ Nuttall, M. and T.V. Callaghan, 2000. The Arctic: Environment, People, Policy. Plate 14. Harwood Academic Publishers, 647p.
- ↑ Skree, O., R. Baxter, R.M.M. Crawford, T.V. Callaghan and A. Fedorkov, 2002. How will the tundra-taiga interface respond to climate change? Ambio Special Report, 12:37–45.
- ↑ Cramer,W., 1997. Modeling the possible impact of climate change on broad-scale vegetation structure: examples from northern Europe. Ecological Studies, No. 124, pp. 1312–1329. Springer.
- ↑ Skree, O., R. Baxter, R.M.M. Crawford, T.V. Callaghan and A. Fedorkov, 2002. How will the tundra-taiga interface respond to climate change? Ambio Special Report, 12:37–45.
- ↑ Vlassova,T.K., 2002. Human impacts on the tundra-taiga zone dynamics: the case of the Russian lesotundra. Ambio Special Report, 12:30–36.