Modeling atmospheric dispersion of pollutants
Contents
- 1 Introduction Air pollution is one of the major environmental challenges facing humankind today. It is generally measured in terms of mass or volume of the species (pollutants) present in unit volume of air (Modeling atmospheric dispersion of pollutants) . It is one of the major causes of respiratory diseases like asthma, bronchitis and lung cancer. There are different kinds of pollutants, some of the most common ones being carbon monoxide, carbon dioxide, oxides of nitrogen and sulfur, etc. Pollutants are generally generated in urban areas (mostly from vehicular traffic) and in industrial regions. Once they are generated, they get transported in the atmosphere by moving air. In general, their movement is governed by a host of factors including meteorological conditions, topography of the local region and the nature of the pollutants themselves. Atmospheric dispersion refers to the behavior of these pollutant particles in the turbulent flows in the atmosphere.
- 2 Meteorological factors influencing dispersion
- 3 Modeling turbulent dispersion
- 4 See also
- 5 Further Reading
Introduction Air pollution is one of the major environmental challenges facing humankind today. It is generally measured in terms of mass or volume of the species (pollutants) present in unit volume of air (Modeling atmospheric dispersion of pollutants) . It is one of the major causes of respiratory diseases like asthma, bronchitis and lung cancer. There are different kinds of pollutants, some of the most common ones being carbon monoxide, carbon dioxide, oxides of nitrogen and sulfur, etc. Pollutants are generally generated in urban areas (mostly from vehicular traffic) and in industrial regions. Once they are generated, they get transported in the atmosphere by moving air. In general, their movement is governed by a host of factors including meteorological conditions, topography of the local region and the nature of the pollutants themselves. Atmospheric dispersion refers to the behavior of these pollutant particles in the turbulent flows in the atmosphere.
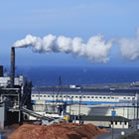
Atmospheric dispersion occurs over a wide range of horizontal length scales. Traditionally, it has been broadly classified as macroscale, mesoscale and microscale processes. The horizontal length scales associated with the macroscale processes are above 1000 kilometers (km), microscale phenomena have horizontal length scales less than 5 km while in between the two lie the mesoscale events. The pollutants, while getting dispersed rarely move above the troposphere, which is the lowest level of the Earth's atmosphere (Atmosphere layers). The troposphere is characterized by a nearly constant rate of decrease in temperature, known as the adiabatic lapse rate and it extends up to an altitude of 9 to 16 km from the ground. The lowest part of the troposphere is known as the planetary boundary layer (PBL); this region is directly influenced by the Earth's surface in the form of frictional drag (from topology and obstacles like buildings etc.), solar heating (radiation) and evapotranspiration. The PBL may extend up to 100 m to 3 km from the ground. Almost all the pollutants (except from aircraft and rockets) are released in this layer, so this is very important for short range pollutant transport. Dispersion in the microscale range almost always takes place wholly in the PBL.
Meteorological factors influencing dispersion
Some of the most important parameters that play a leading role in the dispersion of pollutants in the atmosphere are wind velocity, atmospheric turbulence, stability, temperature, humidity, etc. The pollutants get transported along the direction of wind. But it is the atmospheric turbulence that determines the lateral and vertical spread of the pollutants. Stability assumes a critical role in determining the amount of turbulence in the atmosphere and thus directly affects the level of dispersion.
Turbulence
Turbulence is a phenomenon associated with fluid flows. Fluid motion is governed by the Navier Stokes equations. They comprise a set of coupled, non-linear partial differential equations. They are the momentum equations (one each for the three components of velocity) and the continuity equation that conserves total mass. Furthermore, there is one equation each for temperature and any additional species to predict their transport. The inherent non-linearity in the equations gives rise to extremely complicated behavior of the flow solution that is known as turbulence. There are no known analytical solutions to the Navier Stokes equations except for a few simplified cases. One has to resort to numerical techniques to make some progress.
Turbulent flows are characterized by vortical motions and high rates of dissipation. The vortical motion causes increased dispersion and strong rates of mixing. High dissipation rates contribute to substantial increase in pressure drop across flows in internal channels and also result in high drag across obstacles in flows.
The turbulence in the atmosphere is the reason behind the dispersion of pollutants. If there were no turbulence, the pollutants would have just followed the streamlines of mean wind velocities, so point sources of pollutants would have given rise to long lines of pollutant transport with minimal mixing (due to molecular viscosity) in the lateral directions.
Modeling and simulation of turbulent flows
The Navier Stokes are the governing equations of fluid mechanics. A complete solution of these equations with the correct initial and boundary conditions would exactly predict the flow field including the turbulence. This approach, known as the Direct Numerical Simulation (DNS) has been followed by many fluid dynamicists but huge computational resources are required. Still, DNS remains a very popular tool for detailed understanding of simple flows of academic interest. But it is not feasible to perform these massive computations for practical and industrial problems. Some level of turbulence modeling has to be introduced to alleviate this problem. In general, the higher the level of modeling involved, the greater is the possibility of generating erroneous results, but with skillful use of cleverly developed and carefully calibrated turbulence models, it is possible to obtain engineering results of a wide variety of problems within reasonable accuracy.
Large Eddy Simulation (LES) is a technique that is being increasingly used nowadays both in industry and in academia. Here, the large scales in the flow field are fully resolved numercially while the smaller scales are modeled. The rationale behind modeling the smaller scales (known as sub-grid scale (SGS) modeling) is that they are more universal and do not depend too much on the exact nature of the mean flow; this makes them more amenable to modeling. Some of the more polular SGS models are the Smagorinsky and the dynamic models.
Currently, the most widely used turbulence modeling technique in the industry is the Reynolds Averaged Navier Stokes (RANS) approach. In this method, the Navier Stokes equations are ensemble-averaged and the resulting RANS equations are solved numerically. However, due to the averaging, these equations contain additional unknown terms known as the Reynolds Stress terms that have to be modeled. The exact equations of these Reynolds stresses can be derived but they contain even more additional unknown terms and this is the well known turbulence closure problem. Closure of these terms can be done at different levels of complexity giving rise to a complete hierarchy of turbulence models. The simplest ones construct constitutive relations for the Reynolds stress terms by means of turbulent viscosity. Closure of this turbulence viscosity can be done in different ways of varying complexity starting from pure algebraic zero-equation models to one-equation (e.g. Spalart-Almaras model) and two-equation (e.g. k-ε, k-ω etc.) models that require one and two partial differential equations respectively. More sophisticated models are the second moment closure (SMC) models that solve separate partial differential equations for each of the Reynolds Stresses, Reynolds scalar fluxes and variance in most cases.
Atmospheric stability
Atmospheric stability is related to the vertical profile of density in the atmosphere. Spatial variation of temperature and specific humidity leads to a corresponding variation of density, which is known as density stratification. If cooler (and hence heavier) parcels of air reside above hotter (and hence lighter) parcels, then there is always a tendency of the heavier parcels to move down and the lighter parcels to move up. This kind of stratification is unstable and the associated turbulence is also higher. Stable stratification is a result of lighter parcels residing on top of heavier parcels and is characterized by low turbulence. Uniform density of air results in neutral stratification. Stability plays a direct role in the amount of turbulence present in the atmosphere and therefore significantly affects atmospheric dispersion.
Modeling turbulent dispersion
Modeling turbulent dispersion in the atmosphere is extremely challenging because of the complicated physics mentioned above. Broadly, there are two different modeling approaches – the Eulerian and the Lagrangian techniques. The fluid motion is mostly defined by equations in the Eulerian frame where the fundamental parameters like velocity, pressure and density of the fluid are considered at fixed points in space. The alternate route is the Lagrangian technique where these parameters are considered at each individual fluid particle as it moves along its trajectory. In general, the Eulerian approach will give rise to partial differential equations as the variation of the flow quantities occur in both space and time, while the Lagrangian route will result in ordinary differential equations as the parameters will vary only in time. In dispersion modeling, the flow-field is assumed to be known and the concentration field is required to be computed.
The pollutant source is specified as an input in most pollutant dispersion problems. In general, there may be a large number of sources that may vary in size and shape (e.g. point, line, area and volume sources). However, a source of any shape and size can be modeled as a collection of suitably chosen points. Moreover, the principle of superposition is usually invoked which essentially means that computations can be carried out for each source individually and subsequently the results can be added to get the concentration field for the combined effect of all the sources. Therefore, the point can be regarded as the most fundamental form and a lot of research has focussed on this form of source. However, if the concentration field due to the different sources interact with each other (as in chemical reactions), the assumption of superposition can no longer be valid.
Analytical models of dispersion
There are some analytical models that are widely used especially for regulatory purposes mainly because of their simplicity. Most of these belong to the category of Gaussian Plume diffusion models. The concentration field predicted by these models show Gaussian distribution. It has a theoretical basis in the sense that both Eulerian and Lagrangian forms of the governing equations in extremely simplified cases give solutions of this form. The solutions are valid for instantaneous point sources and continuous point and line sources. The main assumptions associated with these models are uniform, steady flow with homogeneous turbulence. In general, these are not met in actual atmospheric flows and hence more sophisticated models are required.
Eulerian approach towards describing turbulent dispersion
In this approach, the exact governing equation is a transport equation of a scalar (pollutant concentration in this case). Any level of modeling for turbulence will give rise to unknown terms due to Reynolds scalar fluxes. It is these terms that result in high rates of dispersion for the scalar. Investigators in the atmospheric sciences community have widely used gradient transport theories to close the scalar fluxes. These theories, also known as K theories, advocate the use of turbulent diffusivities to address the turbulent scalar diffusion. Further simplifications can be introduced to make the diffusivity isotropic and constant. Analytical solutions exist for these cases making the approach very attractive. Anistropic and some simple, prescribed one-dimensional (in the vertical direction) forms of diffusivities can also give rise to analytical solutions and fairly accurate predictions in some cases. However, in order to get meaningful solutions in complex flows, more complicated forms of diffusivities have to be incorporated in the scalar transport equation, thereby necessitating the use of numerical techniques to solve the equations. Often, when one resorts to numerical simulation, the fluid flow equations along with the associated turbulence models are also solved. The two equation and other lower forms of turbulence models generate eddy viscosities for momentum. Scalar diffusivities are then evaluated by adding a constant value of turbulent Prandtl number as a factor. The more sophisticated and expensive second moment closure models solve separate equations for the Reynolds stresses and the Reynolds scalar fluxes. The unsteady, three-dimensional large-eddy-simulation (LES) currently remains the most expensive and accurate technique for predicting dispersion in atmospheric flows and generally requires the use of large supercomputers.
Lagrangian approach towards describing turbulent dispersion
This approach is generally regarded as more fundamental. Numerical modeling by this technique in most cases assumes the particles that are released at sources to be exactly the same in size, shape and chemical composition as any other fluid particle in the flow field. However, these particles coming out from the sources are viewed as tagged and the study of how they move in the atmosphere comprises the dispersion modeling effort. There are two broad ways of doing the simulation. One is to do a complete and detailed numerical computation by the Eulerian approach like the LES to obtain both the mean and the turbulent velocities in the entire flow field and use these values to compute the Lagrangian trajectories of the tagged particles. This technique, due to its higher accuracy, has been followed by some researchers but it is prohibitively expensive, entailing the use of very powerful workstations and supercomputers. The other, more popular method needs only some mean values as input and calculates the turbulent, fluctuating values by use of a Lagrangian Stochastic Model (LSM). In general, the LSM is actually a set of stochastic differential equations (SDE's), defining the turbulent velocities and in some cases the turbulent temperature. The mean values that are required as input can be obtained from simple experimental observations, empirical relations or some relatively inexpensive Eulerian RANS models. The LSM is the heart of the Lagrangian technique and in most cases the accuracy of the predictions depends wholly on the sophistication of the LSM. These models have progressively developed over the years from the simple models for homogeneous, isotropic turbulence to more complicated ones that take turbulence anisotropy, inhomogeneity and stratification into account. Recently, there have been attempts to obtain direct mathematical relationships with the sophisticated turbulence models like the SMC, resulting in enhanced physics-capturing capabilities and sophistication of the LSM's.
See also
- Air pollution
- Air pollution dispersion modeling
- Air pollution dispersion terminology
- Anthropogenic and natural air pollution emissions
Further Reading
- Durbin, P.A. and Pettersson Reif, B.A., 2001. Statistical Theory and Modeling for Turbulent Flows. John Wiley & Sons, Ltd. ISBN: 0471497444
- Pal Arya, S., 1999. Air Pollution Meteorology and Dispersion. Oxford University Press. ISBN: 0195073983
- D.Bruce Turner, 1994. Workbook of atmospheric dispersion estimates: an introduction to dispersion modeling. CRC Press. ISBN:1-56670-023-X
- Milton Beychok, 2005. Fundamentals of Stack Gas Dispersion. 4th Edition. Self-published. ISBN:0-9644588-0-2