Recent and projected changes in arctic species distributions and potential ranges
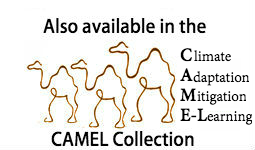
This is Section 7.3.5 of the Arctic Climate Impact Assessment
Lead Author: Terry V. Callaghan; Contributing Authors: Lars Olof Björn, F. Stuart Chapin III,Yuri Chernov,Torben R. Christensen, Brian Huntley, Rolf Ims, Margareta Johansson, Dyanna Jolly Riedlinger, Sven Jonasson, Nadya Matveyeva,Walter Oechel, Nicolai Panikov, Gus Shaver; Consulting Authors: Josef Elster, Heikki Henttonen, Ingibjörg S. Jónsdóttir, Kari Laine, Sibyll Schaphoff, Stephen Sitch, Erja Taulavuori, Kari Taulavuori, Christoph Zöckler
Paleoecological research (Section 7.2 (Recent and projected changes in arctic species distributions and potential ranges)) and observations over many decades demonstrate that the geographic ranges of terrestrial species in general are well correlated with bioclimatic variables. Furthermore, the strength of these relationships is independent of trophic level[1]. Major climate-related species distributions at the large scale include the limit of trees, which is associated with the isoline for mean July air temperatures of about 10 °C[2] (as discussed in [3]) and soil temperature of 7 °C[4], and the limit of woody plants such as dwarf shrubs that are one indicator of the boundary of the polar desert biome[5]. Such relationships suggest that species distributions at the macrogeographical and landscape scale are very likely to change as temperature changes. This section assesses the effects of climate change on recent changes in species distributions and those projected to occur in the future.
Contents
Recent changes (7.3.5.1)
Indigenous knowledge projects have documented recent changes in the ranges of caribou in relation to changes in weather, based on hunters’ understanding of how environmental conditions affect seasonal caribou distribution patterns[6]. Hunters’ explanations of caribou distributions may provide indications of potential range changes given projected climate change. For example, in the El Niño year of 1997–1998, several thousand Porcupine Caribou overwintered on the Yukon Coast in arctic Canada. Hunters in Aklavik, Northwest Territories, explained this phenomenon in terms of the Beaufort Sea ice pack, which was farther from the Yukon North Slope than in most years, resulting in warmer coastal temperatures and thus more abundant forage for caribou. In July 1997, as the caribou moved into Canada from their Alaskan calving grounds, several large groups remained on the coast, taking advantage of the rich forage opportunities. A mild autumn and the lack of icing events that push the caribou south for the winter kept the caribou in the area into October, as the animals could continue to access summer forage. The herd that remained on the coast for the winter was reported to be in better condition than the herd wintering in the usual locations.
Indigenous knowledge has also documented recent changes in the ranges of other animals in relation to changes in the weather. In the Canadian Arctic, Inuit in communities such as Baker Lake have reported insects previously associated with areas south of the treeline[7]. In more western regions, there have been more frequent sightings of "mainland ducks" such as pintail ducks (Anas acuta) and mallard (A. platyrhynchos[8]).
Working in the Canadian Arctic using a conventional scientific approach, Morrison et al.[9] summarized the trends in population data for breeding waders, and found that almost all arctic-breeding species were declining. The reasons for the trends were not always clear and were probably of multiple origins. Long-term monitoring in Finland has shown a substantial decline in the populations of many arctic and subarctic bird species over the past 20 years[10], but the trend in bird populations is not always negative. Zöckler et al.[11] found that almost half of the arctic-breeding, long-distance migrants studied are presently in decline. For many species there are still insufficient data available, and only a few species (8%) show an increasing trend. In most cases, it is not easy to correlate trends with climate change. As the trends in some species are different outside and inside the Arctic, there is an indication that factors of a more global nature are involved. An example is the drastic decline of the ruff (Philomachus pugnax) in almost all breeding sites outside the Arctic in contrast to their stable or even increasing populations in some (but not all) northern arctic areas[12]. This coincides with the recent northern expansion of other wet-grassland waders, such as the common snipe (Gallinago gallinago) in the Bolshemelzkaya tundra[13], the black-tailed godwit (Limosa limosa), and the northern lapwing (Vanellus vanellus) in northern Russia concomitant with a northward expansion of agriculture including sown meadows[14]. Several other bird species have recently been recorded in more northerly locations in the Arctic[15], suggesting that some species are shifting their distribution in response to alteration of habitats by climate change. The emerging picture is that the ruff is being forced to retreat to its core arctic habitats owing to the effects of global climate change in combination with increasing nutrient enrichment on the quality of wet grassland habitats[16].
A recent global meta-analysis of plants claims that a climate change signal has been identified across natural ecosystems[17]. Range shifts of plants averaging 6.1 kilometers (km) per decade toward the poles and 6.1 meters (m) per decade in altitude have been identified in response to a mean advancement of spring (initiation of greening) by 2 to 3 days per decade. Although some northern treeline data were included in the analysis, little information was available for arctic ecosystems.
Projected future changes in species distributions (7.3.5.1)
Models of species–climate response surfaces based upon correlations between species ranges and bioclimatic variables are able to simulate the recently observed range changes of at least some species of birds[18] and butterflies[19]. Related studies have shown that, at least in the case of butterflies, the extent to which species have realized their projected range changes over the last 30 to 50 years is strongly related to their degree of habitat restriction: generalist species are much more able to achieve the projected range expansions than are specialist species[20].
Such models[21] project future ranges of arctic species that are often markedly reduced in spatial extent compared to the species’ present ranges. The range limits of boreal and temperate species shift poleward in response to the same future climate scenarios. However, the large magnitude of the shifts in projected range margins results in potential reductions in the ranges of many boreal species because they are limited to the north by the Arctic Ocean.
The extent to which arctic plant species experience the rapid range reductions simulated by such models will depend principally upon two factors. First, such reductions are likely to happen most rapidly in species that experience some physiological constraint at their southern range margin, for example, the winter thermal constraint postulated for cloudberry[22] or the summer thermal constraints postulated for the great skua (Catharacta skua[23]). Species whose southern range margin is determined by biotic interactions are likely to experience less rapid range reductions. Second, such reductions are very likely to happen more rapidly where the northward migration of boreal or temperate species is not limited either by habitat availability or propagule (dispersal stage of a plant or animal, such as fertilized eggs, larvae, or seeds) dispersal. "Fugitive" species of the early successional communities that characteristically follow disturbance of the boreal forests have the required dispersal ability to achieve rapid poleward range expansions. Unless other factors (e.g., herbivore pressure or a lack of microsites for successful seedling establishment) exclude them, these species are likely to extend into the Arctic rapidly, forming transient ecosystems that will persist until the arrival of the more slowly expanding late-successional boreal species.
Loss of habitat is a particularly important possibility that would constrain species ranges. The most dramatic change in habitat for many water birds is the projected loss of tundra habitat, which varies between 39 and 57% by the end of the 21st century[24]. Vegetation models[25] combined with maps of water-bird distributions show a large variation in the impact of projected vegetation changes on 25 selected species[26]. Vegetation scenarios derived from the HadCM2GSa1 model project that 76% of tundra bean goose (Anser fabalis rossicus/serrirostris) habitat will be affected by the alteration of tundra vegetation, while only 5% of sanderling habitat will be affected[27]. However, the sanderling, similar to many other high-arctic breeders, might be affected even more strongly, as southern tundra habitat types are projected to replace their specific high-arctic habitats. Whereas the more southerly breeding species can shift northwards, it is likely to be increasingly difficult for high-arctic breeders to compete. For two of the three water-bird species that are considered globally threatened, namely the red-breasted goose (Branta ruficollis) and the spoon-billed sandpiper (Eurynorhynchus pygmaeus), 67 and 57% of their current breeding range is projected to change from tundra to forest, respectively (Table 7.7). This additional loss of habitat is likely to place these two species at a higher risk of extinction. The emperor goose (Anser canagicus), already in decline and with 54% of its small range projected to be affected, is highlighted as needing further conservation attention.
Table 7.7. Loss of breeding area habitat projected by two different general circulation models for arctic water-bird species, and their globally threatened status (based on [28]). | ||||
---|---|---|---|---|
Loss of habitat (%)a | ||||
HadCM2GSa1b | UKMOc | Red Listd | ||
Tundra bean goose | Anser fabalis rossicus/serrirostris | 76 | 93 | |
Red-breasted goose | Branta ruficollis | 67 | 85 | VU |
Spoon-billed sandpiper | Eurynorhynchus pygmaeus | 57 | 57 | VU/EN |
Emperor goose | Anser canagicus | 54 | 54 | ! |
Ross’s gull | Rhodostethia rosea | 51 | 73 | |
Red-necked stint | Calidris ruficollis | 48 | 68 | |
Sharp-tailed sandpiper | Calidris acuminata | 46 | 74 | |
Little stint | Calidris minuta | 45 | 65 | |
Curlew sandpiper | Calidris ferruginea | 41 | 70 | |
Pectoral sandpiper | Calidris melanotos | 38 | 60 | |
Dunlin | Calidris alpina | 36 | 58 | |
White-fronted goose | Anser albifrons | 36 | 57 | |
Long-billed dowitcher | Limnodromus scolopaceus | 31 | 54 | |
Great knot | Calidris tenuirostris | 31 | 42 | |
Lesser white-fronted goose | Anser erythropus | 28 | 29 | VU |
Barnacle goose | Branta leucopsis | 21 | 27 | |
Western sandpiper | Calidris mauri | 19 | 21 | |
Brent goose | Branta bernicla | 16 | 44 | |
Red knot | Calidris canutus | 16 | 33 | |
Greater snow goose | Anser caerulescens | 14 | 46 | |
Canada goose | Branta canadensis | 13 | 22 | |
Pink-footed goose | Anser brachyrhynchus | 10 | 10 | |
Sanderling | Calidris alba | 5 | 25 | |
aValue could be substantially higher as unclassified areas in these analyses may contain different tundra types; bmoderate warming; cextreme warming; dVU=vulnerable as a globally threatened species[29], EN=suggested for upgrading to endangered as a globally threatened species,!=suggested for inclusion in the Red List. |
Geographic ranges of plants
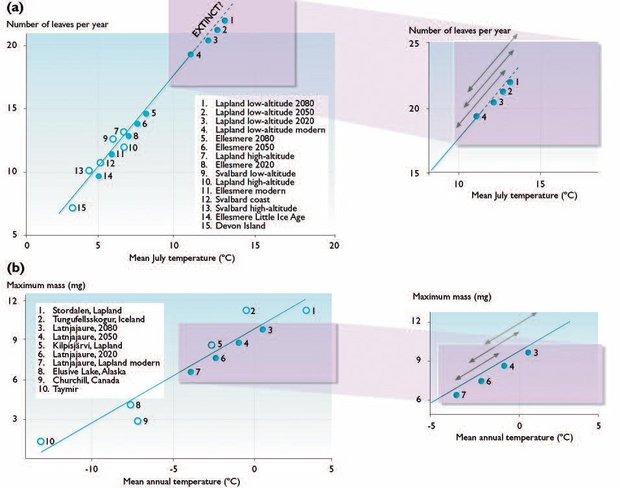
Strong relationships between growth and temperature in the circumpolar ericaceous dwarf shrub Cassiope tetragona and the feather moss Hylocomium splendens can be used to model range changes. The growth of C. tetragona is strongly related to mean July temperature[31] and that of H. splendens is related to mean annual temperature[32] throughout their northern ranges (Fig. 7.15a). Mean July and mean annual temperatures are to some extent representative of latitude, as they decrease toward the north. The natural climatic warming from the beginning of the Little Ice Age to the present is the equivalent of only a minor shift in latitude for C. tetragona. On the other hand, projected future warming is likely to produce a greater latitudinal displacement that, at the northern limit of the current ranges of the two species, is very likely to result in a northern range extension (Fig. 7.15a). In contrast, at the southern edge of the ranges, future warming is very unlikely to increase growth beyond the genetic capabilities of the species, and the dynamics of the species in this part of their ranges are very likely to be determined by the responses of competitors to warming. A similar analysis for H. splendens shows how a current alpine population is very likely to resemble a population from a lowland forested area under projected future climate conditions (Fig. 7.15b).
At the landscape scale, plants are distributed in mosaics associated with microhabitats, and the larger-scale latitudinal range changes are very likely to be associated with initial changes in landscape mosaics. Cushion plants and other species characteristic of wind-exposed patches are likely to become restricted in distribution by increased snow cover. In contrast, plants of snow beds might become more restricted if snow duration decreases. Wetland species will become restricted by drying and species in drier areas will become more restricted by increased soil moisture. Plants currently restricted to south-facing slopes and warm springs (to some extent analogues of future warmer habitats and hot spots of biodiversity) north of their main distribution areas are likely to provide an "inoculum" for rapid colonization of surrounding habitats when climate becomes warmer, although they themselves are likely to be displaced from their current niches by less diverse shrub–thicket communities. Examples include orchids, ferns, and herbs in warm springs on West Greenland (although orchids and ferns are unlikely to become widely distributed), ericaceous dwarf shrubs in some inner fjords of Svalbard, and the large shrubs/small trees of the North Slope of Alaska.
Geographic ranges of animals
Often, causes of observed trends in migrant bird population numbers cannot easily be attributed to local, site-related factors within and outside the Arctic, such as drainage, land use change, hunting and persecution by humans, and predation. Even among global factors, climate change is one of an array of drivers, such as eutrophication, often working in synergy with climate change and reinforcing the effect. In addition, migratory birds are also strongly affected by climate change outside of their arctic breeding grounds. Desertification, droughts, and wetland loss; eutrophication of staging and wintering wetlands; changes in land use; and application of chemicals and nutrients on wintering grounds lead to changes in vegetation and biomass on coastal staging and wintering grounds. The effects of sea-level rise on the extent of coastal staging and wintering grounds are very likely to be particularly harmful, and the hunting pressure on wintering waders in certain areas will also reduce bird [[population]s].
There are few studies of the impacts of climate change on migratory species, although recent trends in some species (e.g., arctic geese) are well known[33]. Very little can be concluded from the observed impacts of current climate variability on migratory birds, as existing monitoring programs are few (e.g., [34]) and often began only recently.
An analysis of Hadley Centre spring and summer temperature and precipitation data over the last 50 years, interpolated over the currently known arctic distribution areas of the white-fronted goose (Anser albifrons) and the Taymir population of the red knot (Calidris canutus canutus), demonstrates a significant correlation between the mean June temperature and the percentage of juveniles in the population as a measure of breeding success. The Nearctic population of the red knot (C. c. islandica) and the curlew sandpiper (Calidris ferruginea) breeding on the Taymir Peninsula do not show such a correlation[35]. The HadCM2GSa1 model, forced with a 1% per year increase in atmospheric CO2 concentrations, projects a moderate increase in mean June temperature (by the time CO2 concentrations double) in the Taymir breeding area of the white-fronted goose, which is likely to favor the goose population. The conditions for the Taymir population are projected to be particularly favorable in the period around 2020. However, a considerable initial cooling and lack of warming over present-day values by 2080 in the breeding grounds of the West Greenland goose population is likely to lead to a drop in the size of the fragile Greenland population. Although the ACIA-designated model projections differ from those used in Zöckler and Lysenko[36], possible decreases in temperature in ACIA Region 1 (Section 18.3.1 (Recent and projected changes in arctic species distributions and potential ranges)) are within the range of the projections (Section 7.6 (Recent and projected changes in arctic species distributions and potential ranges), Table 7.14). The Zöckler and Lysenko[37] study must be interpreted in relation to other factors, such as other weather parameters and natural predation that often fluctuates in three- to four-year cycles according to the abundance of the main prey (i.e., the lemming; see Section 7.4.1.4 (Recent and projected changes in arctic species distributions and potential ranges)). Furthermore, hunting by humans, mainly outside of the Arctic, and the effects of climate change outside of the Arctic, in particular sea-level rise, need to be taken into account.
Investigations of the breeding wader population in northeast Greenland for over 30 years showed that spring snow cover is the main factor governing initiation of egg laying in high-arctic waders, such as the red knot and other sandpipers, while June temperature does not appear to be important[38]. In fact, waders breed earlier in the arid but cool far north of Greenland than they do in the "mild", humid southern areas of the high Arctic, where snow cover is much deeper and more extensive. Projections of future climate for northeast Greenland include cooler summers, later snowmelt, and less snow-free space for the arriving waders to feed, which are likely to lead to later breeding and smaller populations. Snow cover must still be considered the prime regulating factor for initiation of egg laying, but temperature – so important for determining invertebrate food availability (Section 8.5.6 (Recent and projected changes in arctic species distributions and potential ranges)) – is important as well when sufficient snow-free habitat is already present.
Although global climate change in synergy with global eutrophication is likely to lead to an increase in biomass in the Arctic, a change in vegetation height and density, and a general change in vegetation structure with shifts in species distribution that are very likely to have an enormous impact on water birds (which are highly dependent on open landscapes and lightly vegetated breeding sites), global climate change and eutrophication are very likely to provide opportunities for other birds with more southerly distributions, such as owls and woodpeckers. Some birds, including most goose species and a few waders, have demonstrated a certain ability to adjust to new and changing habitats[39], but the majority of birds breeding in the high Arctic are likely to be to be pushed to the edge of survival with little habitat left.
Geographic ranges of microorganisms
Studies of geographic ranges of microbes related to extremely cold environments such as the Arctic, and also to climate change, are in their infancy. Unlike plant and animal ecology, soil microbiology still does not have a solution to the central biogeographical problem of whether soil microorganisms are cosmopolitan (widely distributed) or endemic (restricted to one location) species. Until the ranges of species are known, the bacteria that might be threatened by climate change cannot be identified[40].
The prevailing hypothesis for bacterial biogeography is based on the axiom of the Dutch microbiologists Baas-Becking and Beijerinck, who stated, "Everything is everywhere, but the environment selects"[41]. This hypothesis assumes that free-living bacteria are cosmopolitan in their geographic distribution; they are readily disseminated from one location on earth to another by water and air currents or by animal vectors such as birds that migrate between regions. Only recently has it been possible to rigorously test the cosmopolitan distribution of bacteria with unbiased molecular biology approaches.
Studies outside of the Arctic demonstrate that the cyanobacterium Microcoleus chthonoplastes is a cosmopolitan species[42]. Using different molecular biology techniques, Stetter et al.[43] discovered that hyperthermophilic (heat-loving) Archaea isolated from Alaskan oil reservoirs showed a high degree of DNA–DNA reassociation with selected Archaeoglobus, Thermococcus, and Pyrococcus species, and concluded that the species were the same as those from European thermal marine sources. In a separate study, DNA–DNA reassociation of a strain of Archaeoglobus fulgidus isolated from North Sea (North Sea, Europe) crude oil fields showed 100% relatedness to an Archaeoglobus fulgidus strain from Italian hydrothermal systems[44]. These two studies comprise some of the best evidence to date supporting the cosmopolitan hypothesis of Baas-Becking.
However, 3-chlorobenzoate-degrading bacteria isolated from [[soil]s] in six regions on five continents[45] were found to have restricted or unique ranges. Also, plant species have been reported to harbor their own unique symbiotic species of fungi associated with leaves, bark, roots, etc[46], so, by definition, the existence of endemic plants should imply the existence of respective microbial symbionts. Therefore, arctic microbial communities may consist of a mixture of species: some that are endemic and some that are cosmopolitan.
Summary (7.3.5.3)
Monitoring of distribution ranges with a spatial representation as good as for temperate latitudes is not available for the terrestrial Arctic. Indigenous knowledge projects have documented recent changes in caribou ranges in relation to changes in weather. Hunters’ explanations of caribou distributions may provide indications of potential range changes under scenarios of warming temperatures, such as overwintering of caribou in coastal areas during warm winters. Other arctic indigenous observations include insects previously associated with areas south of the treeline and more frequent sightings of "mainland ducks". In contrast, almost all arctic-breeding species are declining. The reasons for the trends are not always clear and probably of multiple origins, although there are suggestions that some species are shifting their distribution in response to alteration of habitats by climate change.
Quantitative monitoring of conspicuous and popular species such as birds and butterflies has demonstrated that many formerly southern species are rapidly approaching arctic regions and some have already entered. Arctic birds, especially arctic-breeding water birds and waders that can be counted on staging and wintering grounds, show mostly declining population trends; some species have declined dramatically. It is likely that these changes result from the combined action of eutrophication and habitat loss on wintering and staging sites as well as concurrent climate change, although separating the relative contributions of these factors is difficult. Based on climate models, dramatic reductions in the populations of tundra birds are projected as a generally warmer climate is likely to increase vegetation height and decrease the Arctic’s landmass.
Species–climate response-surface models are able to simulate the recently observed range changes of at least some species of both birds and butterflies. At least in the case of butterflies, the extent to which species have realized their projected range changes over the last 30 to 50 years is strongly related to their degree of habitat restriction: generalist species are much more able to achieve the projected range expansions than are specialist species. Simulated potential future ranges are often markedly reduced in spatial extent compared to present ranges. The range limits of boreal and temperate species shift poleward but the large magnitude of the shifts in projected range margins results in potential reductions in the ranges of many boreal species because they are limited to the north by the Arctic Ocean. Species that experience some physiological constraint at their southern range margin are likely to be affected sooner than those that are affected by biotic relationships such as competition from immigrant species. Loss of habitat, such as tundra ponds for many arctic birds, is a particularly important possibility that would constrain species ranges. In contrast, plant [[population]s] that are outliers of more southerly regions and restricted to particularly favorable habitats in the Arctic, are likely to spread rapidly during warming. Models of moss and dwarf-shrub growth along latitudinal gradients show considerable potential for range expansion in the north, but considerable uncertainty, in relation to ACIA scenarios of warming.
Most microorganisms detected in northern ecosystems, such as free-living bacteria, are probably cosmopolitan in their geographic distribution and readily disseminated from one location to another, and the environment selects those that can proliferate. However, some species, particularly symbionts with endemic plants, can themselves be candidates for endemic status.
Chapter 7: Arctic Tundra and Polar Desert Ecosystems
7.1 Introduction (Recent and projected changes in arctic species distributions and potential ranges)
7.2 Late-Quaternary changes in arctic terrestrial ecosystems, climate, and ultraviolet radiation levels
7.3 Species responses to changes in climate and ultraviolet-B radiation in the Arctic
7.3.1 Implications of current species distributions for future biotic change
7.3.2 General characteristics of arctic species and their adaptations in the context of changes in climate and ultraviolet-B radiation levels
7.3.3 Phenotypic responses of arctic species to changes in climate and ultraviolet-B radiation
7.3.4 Genetic responses of arctic species to changes in climate and ultraviolet-B radiation levels
7.3.5 Recent and projected changes in arctic species distributions and potential ranges
7.4 Effects of changes in climate and UV radiation levels on structure and function of arctic ecosystems in the short and long term
7.4.1 Ecosystem structure
7.4.2 Ecosystem function
7.5 Effects of climate change on landscape and regional processes and feedbacks to the climate system
7.6 Synthesis: Scenarios of projected changes in the four ACIA regions for 2020, 2050, and 2080
7.7 Uncertainties and recommendations
References
Citation
Committee, I. (2012). Recent and projected changes in arctic species distributions and potential ranges. Retrieved from http://editors.eol.org/eoearth/wiki/Recent_and_projected_changes_in_arctic_species_distributions_and_potential_ranges- ↑ Huntley, B., M.J. Alfano, J.R.M. Allen, D. Pollard, P.C.Tzedakis, J.-L. de Beaulieu, E. Gruger and B. Watts, 2003. European vegetation during Marine Oxygen Isotope Stage-3. Quaternary Research, 59(2):195–212.
- ↑ Brockmann-Jerosch, H., 1919. Baumgrenze und Klimacharakter. Pflanzengeographische Kommission der Schweiz Naturforschenden Gesellschaft, Beiträge zur geobotanischen Landesaufnahmne, Vol 6. Rascher, Zürich.
- ↑ Körner, C., 1999. Alpine Plant Life. Functional Plant Ecology of High Mountain Ecosystems. Springer, 338pp.
- ↑ Körner, C., 1998. A re-assessment of high elevation treeline positions and their explanation. Oecologia, 115:445–459.
- ↑ Edlund, S.A. and B.T. Alt, 1989. Regional congruence of vegetation and summer climate patterns in the Queen Elizabeth Islands, Northwest Territories, Canada. Arctic, 42:3–23.
- ↑ Kofinas, G. with the communities of Aklavik, Arctic Village, Old Crow and Fort McPherson, 2002. Community contributions to ecological monitoring: knowledge co-production in the U.S.-Canada arctic borderlands. In: I. Krupnik and D. Jolly (eds.). The Earth is Faster Now: Indigenous Observations of Arctic Environmental Change, pp. 54–91. Arctic Research Consortium of the United States, Fairbanks, Alaska.
- ↑ Fox, S., 2002. These are things that are really happening: Inuit perspectives on the evidence and impacts of climate change in Nunavut. In: I. Krupnik and D. Jolly (eds.). The Earth is Faster Now: Indigenous Observations of Arctic Environmental Change, pp. 12–53. Arctic Research Consortium of the United States, Fairbanks, Alaska.
- ↑ Riedlinger, D., 2001. Community-based assessments of change: contributions of Inuvialuit knowledge to understanding climate change in the Canadian Arctic. MSc.Thesis. University of Manitoba.
- ↑ Morrison, R.I.G.,Y. Aubry, R.W. Butler, G.W. Beyersbergen, C. Downes, G.M. Donaldson, C.L. Gratto-Trevor, P.W. Hicklin, V.H. Johnston and R.K. Ross, 2001. Declines in North American shorebird populations. Wader Study Group Bulletin, 94:34–38.
- ↑ Väisänen, R.A, E. Lammi and P. Koskimies, 1998. Muuttuva Pesimälinnusto. Otava, Helsinki, 567pp.
- ↑ Zöckler, C., S. Delany and W. Hagemeijer, 2003. Wader populations are declining – how will we elucidate the reasons? Wader Study Group Bulletin, 100:202-211.
- ↑ Zöckler, C., 2002. A comparison between tundra and wet grassland breeding waders with special reference to the Ruff (Philomachus pugnax). Schriftenreihe für Landschaftspflege und Naturschutz 74. Federal Agency for Nature Conservation, Bonn, 115pp.
- ↑ Morozov, V.V., 1998. Distribution of breeding waders in the north-east European Russian tundras. In: H. Hotker, E. Lebedeva, P.S. Tomkovich, J. Gromadzka, N.C. Davidson, J. Evans, D.A. Stroud and R.B.West (eds.). Migration and International Conservation of Waders: Research and Conservation on North Asian, African and European Flyways. International Wader Studies, 10:186–194.
- ↑ Lebedeva, E., 1998.Waders in agricultural habitats of European Russia. In: H. Hotker, E. Lebedeva, P.S. Tomkovich, J. Gromadzka, N.C. Davidson, J. Evans, D.A. Stroud and R.B. West (eds.). Migration and International Conservation of Waders: Research and Conservation on North Asian, African and European Flyways. International Wader Studies, 10:315–324.
- ↑ Zöckler, C., J.H. Mooij, I.O. Kostin, K. Günther and R. Bräsecke, 1997. Notes on the distribution of some bird species on the Taimyr Peninsula. Vogelwelt, 118:329–338.
- ↑ Zöckler, C., 2002. A comparison between tundra and wet grassland breeding waders with special reference to the Ruff (Philomachus pugnax). Schriftenreihe für Landschaftspflege und Naturschutz 74. Federal Agency for Nature Conservation, Bonn, 115pp.
- ↑ Parmesan, C. and G.Yohe, 2003. A globally coherent fingerprint of climate change impacts across natural systems. Nature, 421:37–42.
- ↑ Zöckler, C. and I. Lysenko, 2000. Water Birds on the Edge: First Circumpolar Assessment of Climate Change Impact on Arctic Breeding Water Birds. WCMC Biodiversity Series No. 11. World Conservation Monitoring Centre, Cambridge, 20pp. plus Annex.
- ↑ Hill, J.K., C.D. Thomas and B. Huntley, 1999. Climate and habitat availability determine 20th century changes in a butterfly's range margin. Proceedings of the Royal Society of London: Biological Sciences, 266:1197–1206.– Hill, J.K., C.D. Thomas and B. Huntley, 2003. Modelling present and potential future ranges of European butterflies using climate response surfaces. In: C.L. Boggs, W.B. Watt and P.R. Ehrlich (eds.). Butterflies: Ecology and Evolution Taking Flight, pp. 149–167. University of Chicago Press.– Virtanen, T. and S. Neuvonen, 1999. Performance of moth larvae on birch in relation to altitude, climate, host quality and parasitoids. Oecologia, 120:92–101.
- ↑ Warren, M.S., J.K. Hill, J.A. Thomas, J. Asher, R. Fox, B. Huntley, D.B. Roy, M.G. Telfer, S. Jeffcoate, P. Harding, G. Jeffcoate, S.G. Willis, J.N. Greatorex-Davies, D. Moss and C.D. Thomas, 2001. Rapid responses of British butterflies to opposing forces of climate and habitat change. Nature, 414:65–69.
- ↑ Hill, J.K., C.D. Thomas and B. Huntley, 2003. Modelling present and potential future ranges of European butterflies using climate response surfaces. In: C.L. Boggs, W.B. Watt and P.R. Ehrlich (eds.). Butterflies: Ecology and Evolution Taking Flight, pp. 149–167. University of Chicago Press.– Huntley, B., P.M. Berry, W. Cramer and A.P. McDonald, 1995. Modelling present and potential future ranges of some European higher plants using climate response surfaces. Journal of Biogeography, 22:967–1001.
- ↑ Marks,T.C., 1978. The carbon economy of Rubus chamaemorus L. II. Respiration. Annals of Botany, 42:181–190.– Marks, T.C. and K. Taylor, 1978. The carbon economy of Rubus chamaemorus L. I. Photosynthesis. Annals of Botany, 42:165–179.
- ↑ Furness, R.W., 1990. Evolutionary and ecological constraints on the breeding distributions and behaviour of skuas. In: R. van den Elzen, K.-L. Schuchmann and K. Schmidt-Koenig (eds.). Current Topics in Avian Biology. Proceedings of the International Centennial Meeting of the Deutsche Ornithologen-Gesellschaft, pp. 153–158. Bonn.
- ↑ Harding, R., P. Kuhry, T.R. Christensen, M.T. Sykes, R. Dankers and S. van der Linden, 2002. Climate feedbacks at the tundra-taiga interface. Ambio Special Report, 12:47–55.– Haxeltine, A. and I.C. Prentice, 1996. BIOME3: An equilibrium terrestrial biosphere model based on ecophysiological constraints, resource availability, and competition among plant functional types. Global Biogeochemical Cycles, 10:693–710.
- ↑ Neilson, P.R. and R.J. Drapek, 1998. Potentially complex biosphere responses to transient global warming. Global Change Biology, 4:505–521.
- ↑ Zöckler, C. and I. Lysenko, 2000. Water Birds on the Edge: First Circumpolar Assessment of Climate Change Impact on Arctic Breeding Water Birds. WCMC Biodiversity Series No. 11. World Conservation Monitoring Centre, Cambridge, 20pp. plus Annex.
- ↑ Neilson, P.R. and R.J. Drapek, 1998. Potentially complex biosphere responses to transient global warming. Global Change Biology, 4:505–521.
- ↑ Zöckler, C. and I. Lysenko, 2000. Water Birds on the Edge: First Circumpolar Assessment of Climate Change Impact on Arctic Breeding Water Birds. WCMC Biodiversity Series No. 11. World Conservation Monitoring Centre, Cambridge, 20pp. plus Annex.
- ↑ BirdLife International, 2001. Threatened Birds of Asia: The BirdLife International Red Data Book. N.J. Collar, A.V. Andreev, S. Chan, M.J. Crosby, S. Subramanya and J.A. Tobias (eds.). BirdLife International, Cambridge, UK, 3038pp.
- ↑ Callaghan, T.V., B.A. Carlsson, M. Sonesson and A. Temesvary, 1997. Between-year variation in climate-related growth of circumarctic populations of the moss Hylocomium splendens. Functional Ecology, 11:157–165.– Havström, M., T.V. Callaghan, S. Jonasson and J. Svoboda, 1995b. Little ice age temperature estimated by growth and flowering differences between sub fossil and extant shoots of Cassiope tetragona, an arctic heather. Functional Ecology, 9:650–654.
- ↑ Havström, M., T.V. Callaghan, S. Jonasson and J. Svoboda, 1995b. Little ice age temperature estimated by growth and flowering differences between sub fossil and extant shoots of Cassiope tetragona, an arctic heather. Functional Ecology, 9:650–654.
- ↑ Callaghan, T.V., B.A. Carlsson, M. Sonesson and A. Temesvary, 1997. Between-year variation in climate-related growth of circumarctic populations of the moss Hylocomium splendens. Functional Ecology, 11:157–165.
- ↑ Madsen, J., G. Cracknell and A.D. Fox (eds.), 1999. Goose Populations of the Western Paleoartic: A Review of Status and Distribution. Wetlands International Publications 48. National Environment Research Institute, Ronde, Denmark, 344pp.
- ↑ Soloviev, M.Y., P.S. Tomkovich and N. Davidson, 1998. An international breeding condition survey of arctic waterfowl: progress report. Wader Study Group Bulletin, 87:43–47.
- ↑ Zöckler, C. and I. Lysenko, 2000. Water Birds on the Edge: First Circumpolar Assessment of Climate Change Impact on Arctic Breeding Water Birds. WCMC Biodiversity Series No. 11. World Conservation Monitoring Centre, Cambridge, 20pp. plus Annex.
- ↑ Zöckler, C. and I. Lysenko, 2000. Water Birds on the Edge: First Circumpolar Assessment of Climate Change Impact on Arctic Breeding Water Birds. WCMC Biodiversity Series No. 11. World Conservation Monitoring Centre, Cambridge, 20pp. plus Annex.
- ↑ Zöckler, C. and I. Lysenko, 2000. Water Birds on the Edge: First Circumpolar Assessment of Climate Change Impact on Arctic Breeding Water Birds. WCMC Biodiversity Series No. 11. World Conservation Monitoring Centre, Cambridge, 20pp. plus Annex.
- ↑ Meltofte, H., 2004. pers. comm. National Environment Research Institute, Denmark. Nutti, E., 2004. Karesuando, Sweden.
- ↑ Lugert, J. and C. Zockler, 2001. The bird fauna of the Yakut horse pastures in northeast Siberia. In: B. Gerken and M. Görner (eds.). Neue Modelle zu Maßnahmen der Landschaftsentwicklung mit großen Pflanzenfressern -Praktische Erfahrungen bei der Umsetzung. Natur- und Kulturlandschaft, 4:458–461.
- ↑ Staley, J.T., 1997. Biodiversity: Are microbial species threatened? Current Opinion in Biotechnology, 8:340–345.
- ↑ Beijerinck, M.W., 1913. De infusies en de ontdekking der backteriën. In: Jaarboek van de Koninklijke Akademie v.Wetenschappen. Müller, Amsterdam.
- ↑ Garcia-Pichel, F., L. Prufert-Bebout and G. Muyzer, 1996. Phenotypic and phylogenetic analyses show Microcoleus chthonoplastes to be a cosmopolitan cyanobacterium. Applied and Environmental Microbiology, 62:3284–3291.
- ↑ Stetter, K.O., R. Huber, E. Blochl, M. Kurr, R.D. Eden, M. Fielder, H. Cash and I. Vance, 1993. Hyperthermophilic archaea are thriving in deep North Sea and Alaskan oil reservoirs. Nature, 365:743–745.
- ↑ Beeder, J., R.K. Nilsen, J.T. Rosnes, T. Torsvik and T. Lien, 1994. Archaeoglobus fulgidus isolated from hot North Sea oil field waters. Applied and Environmental Microbiology, 60:1227–1231.
- ↑ Fulthorpe, R.R., A.N. Rhodes and J.M. Tiedje, 1998. High levels of endemicity of 3-chlorobenzoate-degrading soil bacteria. Applied and Environmental Microbiology, 64:1620–1627.
- ↑ Hawksworth, D.L., 1991. The fungal dimension of biodiversity: magnitude, significance, and conservation. Mycological Research, 95:641–655.