Long-term change and variability in surface UV irradiance in the Arctic
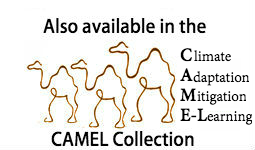
This is Section 5.5 of the Arctic Climate Impact Assessment
Lead Authors: Betsy Weatherhead, Aapo Tanskanen, Amy Stevermer; Contributing Authors: Signe Bech Andersen, Antti Arola, John Austin, Germar Bernhard, Howard Browman,Vitali Fioletov,Volker Grewe, Jay Herman, Weine Josefsson, Arve Kylling, Esko Kyrö, Anders Lindfors, Drew Shindell, Petteri Taalas, David Tarasick; Consulting Authors: Valery Dorokhov, Bjorn Johnsen, Jussi Kaurola, Rigel Kivi, Nikolay Krotkov, Kaisa Lakkala, Jacqueline Lenoble, David Sliney
Several instruments and methods have been used to determine surface UV irradiance in the Arctic. Spectral and broadband radiometers, as well as narrowband multi-filter instruments, are used to measure surface UV irradiance. Quality-controlled measurements of UV irradiance have been available for little more than a decade in the Arctic, with limited spatial coverage. In addition to the direct ground-based UV irradiance measurements, surface UV irradiance can be reconstructed using satellite data, or by using observed total column ozone combined with commonly available meteorological data. In addition, historic UV-B radiation levels can be reconstructed using biological proxies.
Contents
Ground-based Measurements (5.5.1)
Surface UV irradiance measurements have been made in the Arctic for many years[1], and a discernible improvement in the quality of these measurements occurred during the 1990s. Lantz et al.[2] and Bais et al.[3] reported that similarly calibrated spectroradiometers typically differ by less than 5% in the UV-A spectrum, and by 5 to 10% in the UV-B spectrum. However, reliable data have only been available since the 1990s, which is inadequate for long-term trend analyses[4]. Nevertheless, the measured surface UV irradiance time series illustrate the variability of UV radiation and the role of different processes that affect UV irradiance at each of the monitoring sites. The ground-based UV irradiance records are also crucial for validating the indirect methods of estimating UV irradiance.
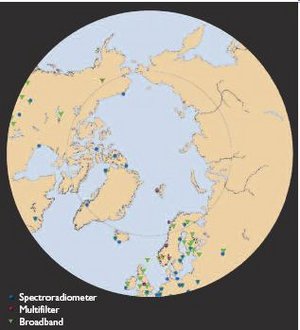
The current network of ground-based UV irradiance measurement stations in the Arctic is shown in Fig. 5.11. Only those installations operated on a regular basis are shown. The measuring instruments fall into three categories: spectroradiometers, multi-filter instruments, and broadband instruments. Spectroradiometers scan radiation in narrow wavelength bands with a typical resolution of 1 nanometer (nm) or less[5]. They provide the greatest measurement accuracy and the spectral information enables versatile data use. However, spectroradiometers are costly and require trained personnel for maintenance and operation. Multi-channel or multi-filter instruments typically consist of several filtered photodetectors that measure radiation in selected wavelength bands[6]. They provide much faster sampling than conventional scanning spectroradiometers, allowing the evaluation of both short- and long-term changes in UV irradiance. Methods have also been developed to reconstruct the high-resolution spectrum from these multi-filter instrument measurements[7]. Broadband instruments collect radiation over a portion of the spectrum and apply a weighting function to the measurements. Many of these instruments are designed to measure the erythemal irradiance. Broadband instruments are comparatively inexpensive and easy to maintain. However, their spectral response often deviates from the one that they are attempting to estimate. Thus, the calibration of broadband instruments depends on solar zenith angle and total column ozone: determining the calibration[8] and maintaining the long-term stability of the broadband instruments can be very demanding[9]. In addition to the instruments described previously, the UV radiation dose (the biological dose rate integrated over time), can be measured using biological UV dosimeters, which directly quantify the biologically effective solar irradiance affecting certain processes by allowing biological systems to act as UV radiation sensors. A number of different sensors have been developed, based on triggers that include direct DNA damage, the inactivation of bacterial spores and bacteriophages, photochemical reactions involving vitamin D photosynthesis, and the accumulation of polycrystalline uracil. Agreement with weighted spectroradiometer measurements has been shown for some of these systems[10].
The Canadian UV-monitoring network includes four arctic measurement sites: Churchill, Resolute, Eureka, and Alert. Monitoring at these sites began in 1992, 1991, 1997, and 1995, respectively. There are reports of increased UV-B radiation levels in the Arctic during six winters in the 1990s[11], but according to Tarasick et al.[12], the maximum UV indices (section 5.1) measured at the Canadian Arctic monitoring sites were 8 in Churchill, 4 in Resolute and Eureka, and 3 in Alert. These values are relatively small compared to the UV indices measured at Canadian monitoring sites outside of the Arctic. However, the maximum observed UV index may not be the best indicator of UV radiation doses received in the Arctic because it does not take into account day length or receptor orientation. Moreover, the Canadian UV measurements imply that snow is a major factor affecting UV irradiance at high latitudes, and may enhance the dose received by a horizontal surface by 40%.
Measurements of spectral UV irradiance at Sodankylä, Finland began in 1990. Lakkala et al.[13] analyzed the Sodankylä data for the period from 1990 to 2001, incorporating corrections for temperature, cosine error, noise spikes, and wavelength shifts. No statistically significant changes in any month were found over the 12-year period, which may be a result of the relatively short period of analysis coupled with the high natural interannual variability of UV irradiance. The lack of a distinct trend over this particular period may also be linked to ozone levels, which decreased in the early 1990s, reached a minimum in the middle of the analysis period, and then increased in the very late 1990s and early 2000s. Arola et al.[14] applied methods to separate the effects of the different factors that affect short- and long-term changes in UV irradiance to the Sodankylä data and found that in some cases, ozone levels accounted for nearly 100% of the short-term variability in monthly mean irradiance, although on average they accounted for about 35% of the variability. The effects of clouds were smaller, accounting for a maximum of 40% and an average of 12% of the short-term irradiance variability. Albedo-related effects were strongest at Sodankylä during the month of May, accounting for a maximum of 21% and an average of 7% of the short-term irradiance variability.
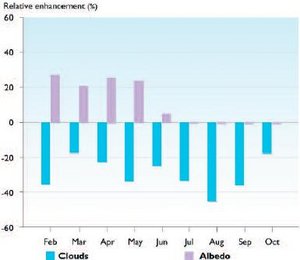
Measurements are also available from Tromsø, Norway and were recently used by Kylling et al.[15] to analyze the effects of albedo and clouds on UV irradiance. The results, shown in Fig. 5.12, indicate that snow increases monthly erythemal UV radiation doses by more than 20% in spring and early summer. The albedo effect at Tromsø is relatively small because the area is influenced by the Gulf Stream, which prevents the adjacent ocean from freezing over. In addition, the terrain around Tromsø is inhomogeneous, consisting of open fjords surrounded by high mountains. Results from a three-dimensional radiative transfer model[16] suggest that the inhomogeneous surface albedo reduces the effective albedo to only 0.55 to 0.60. On specific days, clouds can reduce daily erythemal UV radiation doses at Tromsø by up to 85%. Clouds reduce average monthly erythemal UV radiation doses by 20 to 40% (Fig. 5.12) and this attenuation is fairly constant throughout the year. Multiple scattering between the ground and cloud base links the effects of surface albedo and cloud cover. Attempts to separate the two factors[17] are only approximations.
Spectroradiometer measurements at Barrow, Alaska commenced in January 1991[18]. Increased levels of UV radiation were observed in 1993, which were probably due to low ozone levels related to the injections of aerosols into the stratosphere from the eruption of Mt. Pinatubo[19]. Photochemically induced ozone depletion events at Barrow have primarily occurred during March and April. The most notable case occurred on 18 April 1997, when the daily erythemal UV radiation dose was 36% higher than the climatological average dose. This increase is still small compared to spikes seen in UV radiation measurements at sites affected by the Antarctic ozone hole. By comparing measured and modeled spectra, Bernhard et al.[20] estimated that Barrow’s effective UV albedo (surface albedo for UV wavelengths) during the winter and early spring is 0.85±0.10. The albedo is greater than that of Tromsø because the ocean adjacent to Barrow is frozen until late in the spring, and the treeless tundra surrounding the measurement site is covered by snow until June. The higher albedo leads to a 40 to 55% enhancement of erythemal UV radiation when skies are clear. No statistically significant changes in UV irradiance were found at Barrow for the period from 1991 to 2001[21], for reasons similar to those for Sodankylä. It should be noted that the observed reductions in ozone levels have been much greater in the European and Russian sectors of the Arctic than over Alaska. UV radiation increases at Barrow are therefore less pronounced compared to those measured at the other arctic sites.
Reconstructed Time Series (5.5.2)
In order to assess variations in UV irradiance over longer time periods, various empirical methods for reconstructing UV irradiance data have been developed. These methods are usually based on total column ozone or other commonly available weather data, including global (direct and diffuse) radiation levels. Bodeker and McKenzie[22], for example, presented a model based on total column ozone, broadband radiation measurements, and radiative transfer calculations. Similar methods were developed and used by McArthur et al.[23], Kaurola et al.[24], and Feister et al.[25] for estimating UV irradiance at various locations in Canada and Europe. Gantner et al.[26] estimated clear-sky UV irradiance using an empirically determined statistical relationship between clear-sky UV irradiance and total column ozone. The results obtained with reconstruction methods generally compare well with observations, but there are some sources of uncertainty. For example, accounting for the influence of aerosols on the amount of UV radiation reaching the surface of the earth is difficult, as is accounting for sulfur dioxide from volcanic and anthropogenic sources, although Fioletov et al.[27] reported that sulfur dioxide had a negligible influence on erythemal UV irradiance at 12 out of 13 Canadian and Japanese sites. The importance of examining the homogeneity of the data cannot be overestimated when using long time series of measurements as input data for estimating past UV radiation levels. Likewise, it is of great importance to properly validate the empirical methods against independent measurement data.
Fioletov et al.[28] developed a statistical model using global radiation levels, total column ozone, dew point temperature, and snow cover data to reconstruct past UV irradiance at several Canadian sites, including Churchill. The model is based on previous efforts to estimate UV-A irradiance from pyranometer measurements[29]. Fioletov et al.[30] produced UV irradiance estimates for the period from 1965 to 1997, and found a statistically significant increase in the erythemally weighted UV irradiance (~11% per decade) over the period from 1979 to 1997. This increase follows a reported decline in UV radiation levels from 1965 to 1980. The increase in UV irradiance between 1979 and 1997 is more than twice the increase that would be expected to result from the observed decline in total column ozone, because concurrent changes in surface albedo and cloud conditions have enhanced the increase in surface UV irradiance over this period. Fioletov et al.[31] also examined the frequency of extreme values of UV irradiance at Churchill, and reported that the number of hours when the UV index exceeds five increased from an average of 26 per year during the period 1970 to 1979 to an average of 58 per year during the period 1990 to 1997.
Díaz et al.[32] estimated narrowband (303.030– 307.692 nm) irradiances for Barrow from pyranometer data and satellite-measured total column ozone, and found that spring UV irradiance increased between 1979 and 2000. Lindfors et al.[33] presented a method for estimating daily erythemal UV radiation doses using total column ozone, sunshine duration, and snow depth as input data. They estimated UV radiation doses at Sodankylä for the period from 1950 to 1999, and found a statistically significant March increase (3.9% per decade). April also showed an increasing trend. For both March and April, the trend was more pronounced during the latter part of the period (1979–1999), suggesting a connection to stratospheric ozone depletion. For July, a statistically significant decreasing trend of 3.3% per decade was found, attributed to changes in total column ozone and sunshine duration (defined by the World Meteorological Organization as the time during which direct solar radiation exceeds 120 W/m2). June and August exhibited decreasing trends, although not statistically significant, and trends for May and September were negligible.
In addition to atmospheric observations, biological proxies can be used to estimate past UV irradiance. For example, Leavitt et al.[34] proposed that the past UV radiation environment of freshwater ecosystems could be reconstructed by studying fossil pigments in lake sediments, because some algae and other aquatic organisms produce photoprotective pigments when exposed to UV radiation. However, the longterm variation of underwater UV irradiance is primarily controlled by the amount of dissolved organic matter, which limits the use of fossil pigment sediments for reconstructing surface UV irradiance without ancillary information about dissolved organic matter[35].
Archaeological findings offer further evidence that UV radiation has long been a concern in the Arctic. Goggles found in the ancestral remains of indigenous peoples have thin slits, which allow the wearer to see but limit the amount of sunlight reaching the eyes[36]. The indigenous peoples of the Arctic have historically relied heavily on the environment for their livelihood and well-being, and have therefore been acutely aware of changes in climate-related variables. Fox[37] (2000) documented some of these observations, which include reports of sunburn and increased incidence of snow blindness in populations not normally experiencing these effects. The existence of goggles confirms that arctic peoples have long sought protection from the sun and its glare, but the reports of sunburn and other effects of UV radiation suggest that the recent changes in UV irradiance are unusual when considered in this longer context. This information serves as a useful proxy of UV irradiance over time, and provides firsthand evidence of UV radiation impacts resulting from ozone depletion in the Arctic. The information also helps to corroborate available UV radiation and ozone measurements.
Surface Estimates from Satellite Data (5.5.3)
Estimates of surface UV irradiance derived from satellite data use a radiative transfer model, with input parameters determined from the satellite measurements. In addition to total column ozone, satellite-derived information about clouds, aerosols, and surface albedo is needed to accurately model these parameters, and various sources of data have been used for that purpose[38]. The advantages of satellite measurements include global or near-global spatial coverage and long-term data continuity. However, there are limitations: the best spatial resolution of the currently available data from ozone-monitoring satellite instruments is 40 x 40 kilometers (km) and satellites usually provide only a single overpass per day. Satellite instruments also have difficulty probing the lower atmosphere (Atmosphere layers), where UV-absorbing aerosols or tropospheric ozone can significantly affect surface UV irradiance. Fortunately, neither absorbing aerosols nor tropospheric ozone are major factors affecting UV radiation in the Arctic, with the exception of air masses occasionally transported from lower latitudes (Section 5.4.5 (Long-term change and variability in surface UV irradiance in the Arctic)).
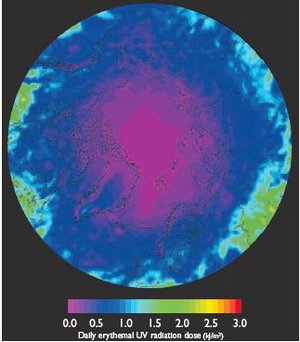
Several studies have assessed the accuracy and limitations of satellite-based UV irradiance estimates by comparing them to ground-based data[39]. For noon irradiance, the root mean square difference between the TOMS-derived estimates and ground-based measurements is of the order of ±25%. Estimating surface UV irradiance in the Arctic is more uncertain than that at lower latitudes: when the ground is snow-covered, the satellite-derived UV irradiance estimates have been systematically lower than the ground-based measurements. The bias originates from underestimates of surface albedo, which is a critical parameter for accurately estimating surface UV irradiance in the Arctic. Some studies have addressed surface albedo variations related to snow and ice cover[40], but none of the proposed methods have completely resolved the issue. Snow or ice cover also complicates the determination of cloud optical depth properties[41], and the radiative transfer models are less accurate at high solar zenith angles. Because of these limitations, the accuracy of current satellite retrieval algorithms is not adequate for monitoring arctic surface UV irradiance, although some information on variations in UV irradiance over time can be inferred. The satellite-derived daily erythemal UV radiation dose in and near the Arctic on 21 March 2000 is shown in Fig. 5.13.
Long-term trends in surface UV irradiance were determined using time series derived from TOMS data. The analyses were performed for clear-sky estimates (i.e., the modulation of surface UV radiation by clouds or aerosols was not considered). The trend analysis methods applied were similar to those used to study long-term changes in total column ozone. The trend models take into account changes in solar activity and oscillations in the atmospheric circulation that affect stratospheric ozone levels. Using TOMS Version 7 ozone and reflectivity data from 1979 to 1992 and the corresponding TOMS surface UV retrieval algorithm, Herman et al.[42] estimated that zonally averaged mean annual surface UV irradiances at 300, 310, and 320 [[nanometer]s] (nm) increased by 15%, 6%, and 2% per decade, respectively, for the latitude band 60º to 70º N. The greatest increases were found in winter and spring. Ziemke et al.[43] studied the spatial distribution of the trends in erythemally weighted surface UV irradiance at northern latitudes using TOMS/Nimbus-7 data and found positive trends exceeding 10% per decade at high latitudes in Eastern Siberia. Unfortunately, the studies based on long-term satellite data did not include the region north of 65º N, and the retrieval algorithm used for the calculations is known to underestimate surface UV irradiance over snow-covered terrain.
Chapter 5: Ozone and Ultraviolet Radiation
5.1. Introduction (Long-term change and variability in surface UV irradiance in the Arctic)
5.2. Factors affecting arctic ozone variability
5.3. Long-term change and variability in ozone levels
5.4. Factors affecting surface ultraviolet radiation levels in the Arctic
5.5. Long-term change and variability in surface UV irradiance
5.6. Future changes in ozone
5.7. Future changes in ultraviolet radiation
5.8. Ozone and Ultraviolet Radiation in the Arctic: Gaps in knowledge, future research, and observational needs
References
Citation
Committee, I. (2012). Long-term change and variability in surface UV irradiance in the Arctic. Retrieved from http://editors.eol.org/eoearth/wiki/Long-term_change_and_variability_in_surface_UV_irradiance_in_the_Arctic- ↑ Hisdal, V., 1986. Spectral distribution of global and diffuse solar radiation at Ny Alesund, Spitzbergen. Polar Research, 5:1–27.-- Stamnes, K., K Henriksen and P. Ostensen, 1988. Simultaneous measurement of UV radiation received by the biosphere and total ozone amount. Geophysical Research Letters, 15:4418–4425.-- Wester, U., 1997. A portable lamp system circulated between Nordic solar UV laboratories. In: B. Kjeldstad, B. Johnsen and T. Koskela (eds.). The Nordic Intercomparision of Ultraviolet and Total Ozone Instruments at Izana, Oct. 1996: Final Report, pp. 25–42. Yliopistopaino, Helsinki.
- ↑ Lantz, K.O., P. Disterhoft, J.J. DeLuisi, E. Early, A. Thompson, D. Bigelow and J. Slusser, 1999. Methodology for deriving clearsky erythemal calibration factors for UV broadband radiometers of the U.S. Central Calibration Facility. Journal of Atmospheric and Oceanic Technology, 16:1736–1752.
- ↑ Bais, A.F., B.G. Gardiner, H. Slaper, M. Blumthaler, G. Bernhard, R. McKenzie, A.R. Webb, G. Seckmeyer, B. Kjeldstad, T. Koskela, P.J. Kirsch, J. Gröbner, J.B. Kerr, S. Kazadzis, K. Leszczynski, D. Wardle, C. Brogniez, W. Josefsson, D. Gillotay, H. Reinen, P. Weihs, T. Svenoe, P. Eriksen, F. Kuik, A. Redondas, 2001. SUSPEN intercomparison of ultraviolet spectroradiometers. Journal of Geophysical Research, 106(D12):12509–12525.
- ↑ Weatherhead, E.C. (Ed.), 1998. Climate change, ozone, and ultraviolet radiation. In: AMAP Assessment Report: Arctic Pollution Issues, pp. 717–774. Arctic Monitoring and Assessment Programme, Oslo.
- ↑ Seckmeyer, G., A. Bais, G. Bernhard, M. Blumthaler, C.R. Booth, P. Disterhoft, P. Eriksen, R.L. McKenzie, M. Miyauchi and C. Roy, 2001. Instruments to measure solar ultraviolet radiation. Part 1: Spectral instruments. Global Atmospheric Watch Publication No. 125,WMO TD No. 1066.World Meteorological Organization.
- ↑ Bigelow, D.S., J.R. Slusser, A.F. Beaubien and J.H. Gibson, 1998. The USDA Ultraviolet Radiation Monitoring Program. Bulletin of the American Meteorological Society, 79:601–615.-- Dahlback, A., 1996. Measurements of biologically effective UV doses, total ozone abundances, and cloud effects with multichannel, moderate bandwidth filter instruments. Applied Optics, 35(33):6514–6521.-- Harrison, L., J. Michalsky and J. Berndt, 1994. Automated multifilter rotating shadow-band radiometer and instrument for optical depth and radiation measurements. Applied Optice, 33:5118–5125.
- ↑ Dahlback, A., 1996. Measurements of biologically effective UV doses, total ozone abundances, and cloud effects with multichannel, moderate bandwidth filter instruments. Applied Optics, 35(33):6514–6521.-- Fuenzalida, H.A., 1998. Global ultraviolet spectra derived directly from observations with multichannel radiometers. Applied Optics, 37(33):7912–7919.-- Min, Q. and L. Harrison, 1998. Synthetic spectra for terrestrial ultraviolet from discrete measurements. Journal of Geophysical Research, 105:17,033–17,039.
- ↑ Bodhaine, B.A., E.G., Dutton, R.L. McKenzie and P.V. Johnston, 1998. Calibrating broadband UV instruments: ozone and solar zenith angle dependence. Journal of Atmospheric and Oceanic Technology, 15:916–926.-- Mayer, B. and G. Seckmeyer, 1996. All-weather comparison between spectral and broadband (Robertson-Berger) UV measurements. Photochemistry and Photobiology, 64(5):792–799.
- ↑ Borkowski, J.L., 2000. Homogenization of the Belsk UV-B series (1976–1997) and trend analysis. Journal of Geophysical Research, 105(D4):4873–4878.-- Weatherhead, E.C., G.C. Tiao, G.C. Reinsel, J.E. Frederick, J.J. DeLuisi, D. Choi and W.K. Tam, 1997. Analysis of long-term behavior of ultraviolet-B radiation measured by Robertson-Berger meters at 14 sites in the United States. Journal of Geophysical Research, 102(D7):8737–8754.
- ↑ Bérces, A., S. Fekete, P. Gáspá, P. Gróf, P. Rettberg, G. Horneck and G. Rontó, 1999. UV dosimeters in the assessment of the biological hazard from environmental radiation. Journal of Photochemistry and Photobiology B-Biology, 53:36–43.-- Furusawa, Y., L.E. Quintern, H. Holtschmidt, P. Koepke and M. Saito, 1998. Determination of erythema-effective solar radiation in Japan and Germany with a spore monilayer film optimized for the detection of UVB and UVA – results of a field campaign. Applied Microbiology and Biotechnology, 50:597–603.-- Munakata, N., S. Kazadzis, A. Bais, K. Hieda, G. Rontó, P. Rettberg and G. Horneck, 2000. Comparisons of spore dosimetry and spectral photometry of solar-UV radiation at four sites in Japan and Europe. Photochemistry and Photobiology, 72:739–745.
- ↑ Fioletov, V.E. and W.F.J. Evans, 1997. The influence of ozone and other factors on surface radiation. In: D.I. Wardle, J.B. Kerr, C.T. McElroy and D.R. Francis (eds.). Ozone Science: a Canadian Perspective on the Changing Ozone Layer, pp. 73–90. Environment Canada Report CARD 97-3.-- Kerr, J.B. and C.T. McElroy, 1993. Evidence for large upward trends of ultraviolet-B radiation linked to ozone depletion. Science, 262(5136):1032–1034.
- ↑ Tarasick, D.W., V.E. Fioletov, D.I., Wardle, J.B. Kerr, J.B. McArthur and C.A. McLinden, 2003. Climatology and trends in surface UV radiation survey article. Atmosphere-Ocean, 41(2) 121–138.
- ↑ Lakkala, K., E. Kyro and T. Turunen, 2003. Spectral UV measurements at Sodankylä during 1990–2001. Journal of Geophysical Research, 108(D19): doi:10.1029/2002JD003300.
- ↑ Arola, A., J. Kaurola, L. Koskinen, A. Tanskanen, T. Tikkanen, P. Taalas, J.R. Herman, N. Krotkov and V. Fioletov, 2003. A new approach to estimating the albedo for snow-covered surfaces in the satellite UV method. Journal of Geophysical Research, 108(D17):4531 doi:10.1029/2003JD003492.
- ↑ Kylling, A., A Dahlback and B. Mayer, 2000a. The effect of clouds and surface albedo on UV irradiances at a high latitude site. Geophysical Research Letters, 27(9):1411–1414.
- ↑ Kylling, A. and B. Mayer, 2001. Ultraviolet radiation in partly snow covered terrain: observations and three-dimensional simulations. Geophysical Research Letters, 27(9):1411–1414.
- ↑ Arola, A., J. Kaurola, L. Koskinen, A. Tanskanen, T. Tikkanen, P.T aalas, J.R. Herman, N. Krotkov and V. Fioletov, 2003. A new approach to estimating the albedo for snow-covered surfaces in the satellite UV method. Journal of Geophysical Research, 108(D17):4531 doi:10.1029/2003JD003492.-- Kylling, A., A. Dahlback and B. Mayer, 2000a. The effect of clouds and surface albedo on UV irradiances at a high latitude site. Geophysical Research Letters, 27(9):1411–1414.
- ↑ Booth, C.R., G. Bernhard, J.C. Ehramjian, V.V. Quang, and S.A. Lynch, 2001. NSF Polar Programs UV Spectroradiometer Network 1999–2000 Operations Report. Biospherical Instruments, San Diego, 219pp.
- ↑ Gurney, K.R., 1998. Evidence for increasing ultraviolet irradiance at Point Barrow, Alaska. Geophysical Research Letters, 25:903–906.
- ↑ Bernhard, G., C.R. Booth and R. McPeters, 2003. Calculation of total column ozone from global UV spectra at high latitudes. Journal of Geophysical Research, 108(D17):4532 doi: 10.1029/2003JD003450.
- ↑ Booth, C.R., G. Bernhard, J.C. Ehramjian, V.V. Quang, and S.A. Lynch, 2001. NSF Polar Programs UV Spectroradiometer Network 1999–2000 Operations Report. Biospherical Instruments, San Diego, 219pp.
- ↑ Bodeker, G.E. and R.L. McKenzie, 1996. An algorithm for inferring surface UV irradiance including cloud effects. Journal of Applied Meteorology, 35:1860–1877.
- ↑ McArthur, L.J.B., V.E. Fioletov, J.B. Kerr, C.T. McElroy and D.I. Wardle, 1999. Derivation of UV-A irradiance from pyranometer measurements. Journal of Geophysical Research, 104(D23): 30139–30151.
- ↑ Kaurola, J., P. Taalas and T. Koskela, 2000. Long-term variations of UV-B doses at three stations in northern Europe. Journal of Geophysical Research, 105(D16):20813–20820.
- ↑ Feister, U., E. Jakel and K. Geridee, 2002. Parameterization of daily solar global ultraviolet radiation Photochemistry and Photobiology, 76(3):281–293.
- ↑ Gantner, L., P. Winkler and U. Kohler, 2000. A method to derive long-term time series and trends of UV-B radiation (1968–1997) from observations at Hohenpeissenberg (Bavaria). Journal of Geophysical Research, 105(D4):4879–4880.
- ↑ Fioletov, V.E., E. Griffioen and J.D. Kerr, 1998. Influence of volcanic sulfur dioxide on spectral UV irradiance as measured by Brewer spectroradiometer. Geophysical Research Letters, 25(10): 1665–1668.
- ↑ Fioletov, V.E., L.J.B. McArthur, J.B. Kerr and D.I. Wardle, 2001. Long-term variations of UV-B irradiance over Canada estimated from Brewer observations and derived from ozone and pyranometer measurements. Journal of Geophysical Research, 106(D19):23,009–32,027.
- ↑ McArthur, L.J.B., V.E. Fioletov, J.B. Kerr, C.T. McElroy and D.I. Wardle, 1999. Derivation of UV-A irradiance from pyranometer measurements. Journal of Geophysical Research, 104(D23): 30139–30151.
- ↑ Fioletov, V.E., L.J.B. McArthur, J.B. Kerr and D.I. Wardle, 2001. Long-term variations of UV-B irradiance over Canada estimated from Brewer observations and derived from ozone and pyranometer measurements. Journal of Geophysical Research, 106(D19):23,009–32,027.
- ↑ Fioletov, V.E., L.J.B. McArthur, J.B. Kerr and D.I. Wardle, 2001. Long-term variations of UV-B irradiance over Canada estimated from Brewer observations and derived from ozone and pyranometer measurements. Journal of Geophysical Research, 106(D19):23,009–32,027.
- ↑ Díaz. S., D. Nelson, G. Deferrari and C. Camilión, 2003. A model to extend spectral and multiwavelength UV irradiances time series: model development and validation. Journal of Geophysical Research, 108(D4): 10.1029/2002JD002134.
- ↑ Lindfors, A.V., A. Arola, J. Kaurola, P. Taalas and T. Svenøe, 2003. Long-term erythemal UV doses at Sodankyla estimated using total ozone, sunshine duration, and snow depth. Journal of Geophysical Research, 108(D16):10.1029/2002JD003325.
- ↑ Leavitt, P.R., R.D. Vinebrooke, D.B. Donald, J.P. Smol and D.W. Schindler, 1997. Past ultraviolet radiation environments in lakes derived from fossil pigments. Nature, 388:457–459.
- ↑ Leavitt, P.R., B.F. Cumming and J.P. Smol, 2003. Climatic control of ultraviolet radiation effects on lakes. Limnology and Oceanography, 48(5):2062–2069.
- ↑ Hedblom, E.E., 1961. Snowscape eye protection. Archives of Environmental Health, 2:685–704.-- Sliney, D.H., 2001. Photoprotection of the eye – UV radiation and sunglasses. Photochemistry and Photobiology, 64:166–175.
- ↑ Fox, S.L., 2000. Arctic climate change: observations of Inuit in the Eastern Canadian Arctic. In: F. Fetterer and V. Radionov (eds.). Arctic Climatology Project, Environmental Working Group Arctic Meteorology and Climate Atlas. U.S. National Snow and Ice Data Center, Boulder. CD-ROM.
- ↑ Herman, J.R., N. Krotkov, E. Celarier, D. Larko and G. Labow, 1999. The distribution of UV radiation at the Earth’s surface from TOMS measured UV-backscattered radiances. Journal of Geophysical Research, 104:12059–12076.-- Krotkov, N.A., J.R. Herman, P.K. Bhartia, V. Fioletov and Z. Ahmad, 2001. Satellite estimation of spectral surface UV irradiance 2. Effects of horizontally homogeneous clouds and snow. Journal of Geophysical Research, 106:11743–11759.-- Li, Z., P. Wang and J. Cihlar, 2000. A simple and efficient method for retrieving surface UV radiation dose rate from satellite. Journal of Geophysical Research, 105:5027–5036.-- Lubin, D., E.H. Jensen and H.P. Gies, 1998. Global surface ultraviolet radiation climatology from TOMS and ERBE data. Journal of Geophysical Research, 103:26061–26091.-- Meerkoetter, R., B. Wissinger and G. Seckmeyer, 1997. Surface UV from ERS-2/GOME and NOAA/AVHRR data: a case study. Geophysical Research Letters, 24:1939–1942.-- Verdebout, J., 2000. A method to generate surface UV radiation maps over Europe using GOME, Meteosat, and ancillary geophysical data, Journal of Geophysical Research, 105:5049–5058.
- ↑ Arola, A., S. Kalliskota, P.N. den Outer, K. Edvardsen, G. Hansen, T. Koskela, T.J. Martin, J. Matthijsen, R. Meerkoetter, P. Peeters, G. Seckmeyer, P. Simon, H. Slaper, P. Taalas and J. Verdebout, 2002. Four UV mapping procedures using satellite data and their validation against ground-based UV measurements. Journal of Geophysical Research, 107, (D16):4310 doi:10.1029/2001JD000462.-- Chubarova, N.Y., A.Y. Yurova, N.A. Krotkov, J.R. Herman and P.K. Bhartia, 2002. Comparisons between ground based measurements of broadband UV irradiance (300–380 nm) and TOMS UV estimates at Moscow for 1979–2000. Optical Engineering, 41(12):3070–3081.-- Fioletov, V.E., J.B. Kerr, D.I. Wardle, N. Krotkov and J.R. Herman, 2002. Comparison of Brewer UV irradiance measurements with TOMS satellite retrievals. Optical Engineering, 41(12): 3051–3061.-- Kalliskota, S., J. Kaurola, P. Taalas, J.R. Herman, E.A. Celarier and N. Krotkov, 2000. Comparison of daily UV doses estimated from Nimbus 7/TOMS measurements and ground-based spectroradiometric data. Journal of Geophysical Research, 105:5059–5067.-- McKenzie, R.L., G. Seckmeyer, A.F. Bais, J.B. Kerr and S. Madronich, 2001b. Satellite retrievals of erythemal UV dose compared with ground-based measurements at northern and southern midlatitudes. Journal of Geophysical Research, 106(D20): 24051–24062.-- Slusser, J., W. Gao and R. McKenzie, 2002. Advances in UV ground- and space-based measurements and modeling. Optical Engineering, 41(12):3006–3007.-- Wang, P., Z. Li and J. Cihlar, 2000. Validation of an UV inversion algorithm using satellite and surface measurements. Journal of Geophysical Research, 105:5037–5048.
- ↑ Arola, A., J. Kaurola, L. Koskinen, A. Tanskanen, T. Tikkanen, P. Taalas, J.R. Herman, N. Krotkov and V. Fioletov, 2003. A new approach to estimating the albedo for snow-covered surfaces in the satellite UV method. Journal of Geophysical Research, 108(D17):4531 doi:10.1029/2003JD003492.-- Lubin, D. and E. Morrow, 2001. Ultraviolet radiation environment of Antarctica. 1. Effect of sea ice on top-of-atmosphere albedo and on satellite retrievals. Journal of Geophysical Research, 106: 33453–33461.-- Tanskanen, A., A. Arola and J. Kujanpää, 2003. Use of the moving time-window technique to determine surface albedo from TOMS reflectivity data. In: Proceedings of the International Society for Optical Engineering Vol. 4896.
- ↑ Krotkov, N.A., J.R. Herman, P.K. Bhartia, V. Fioletov and Z. Ahmad, 2001. Satellite estimation of spectral surface UV irradiance 2. Effects of horizontally homogeneous clouds and snow. Journal of Geophysical Research, 106:11743–11759.
- ↑ Herman, J.R., P.K. Bhartia and J. Ziemke, 1996. UV-B increases (1979–1992) from decreases in total ozone. Geophysical Research Letters, 23(16):2117–2120.
- ↑ Ziemke, J.R., S. Chandra and J. Herman, 2000. Erythemally weighted UV trends over northern latitudes derived from Nimbus 7 TOMS measurements. Journal of Geophysical Research, 105(D6):7373–7382.