Ultraviolet radiation effects on freshwater ecosystems in the Arctic
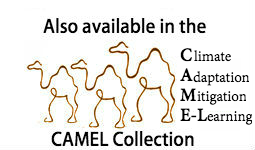
This is Section 8.6 of the Arctic Climate Impact Assessment
Lead Authors: Frederick J.Wrona,Terry D. Prowse, James D. Reist; Contributing Authors: Richard Beamish, John J. Gibson, John Hobbie, Erik Jeppesen, Jackie King, Guenter Koeck, Atte Korhola, Lucie Lévesque, Robie Macdonald, Michael Power,Vladimir Skvortsov,Warwick Vincent; Consulting Authors: Robert Clark, Brian Dempson, David Lean, Hannu Lehtonen, Sofia Perin, Richard Pienitz, Milla Rautio, John Smol, Ross Tallman, Alexander Zhulidov
Ultraviolet radiation is the most photochemically reactive wavelength of solar energy reaching the surface of the earth, and has a broad range of effects on aquatic biogeochemistry, biota, and ecosystems. As a result of anthropogenic impacts on the atmosphere of the earth, UV radiation exposure in arctic environments is changing substantially. Although anthropogenic emissions of ozone-depleting substances have declined since the ratification of the Montreal Protocol and its amendments, future levels of ozone and UV radiation in the Arctic are uncertain, depending not only on continued compliance with the Protocol and changes in legislation, but also on climate change effects on temperatures and trace gases (e.g., Sections 5.6.2, 7.5, and 8.4.4.4 (Ultraviolet radiation effects on freshwater ecosystems in the Arctic)). This section provides an overview of how underwater UV radiation exposure is linked to climate, followed by a discussion of general principles concerning UV radiation impacts in aquatic ecosystems (including natural protection mechanisms) and a systematic analysis of potential UV radiation impacts on arctic freshwater habitats.
Contents
Climate effects on underwater ultraviolet radiation exposure (8.6.1)
To understand the overall impact of changes in UV radiation levels, the synergistic and antagonistic processes resulting from climate change have to be considered since they have the potential to modify the underwater UV radiation regime and consequently the stress on aquatic organisms. Climate change is very likely to be accompanied by shifts in biological UV radiation exposure in arctic river, lake, and wetland environments via three mechanisms[1]: changes in stratospheric ozone levels, changes in snow- and ice-cover duration, and changes in the colored materials dissolved in natural waters that act as sunscreens against UV radiation.
Although it is projected that the downward trends in ozone levels are likely to reverse in the near future as a consequence of reduced anthropogenic emissions of chlorofluorocarbons (CFCs) and related compounds, some of the longer-lived ozone-depleting substances are still accumulating in the stratosphere and climate change is likely to prolong the effects of depletion. Temperature increases in the troposphere are projected to be accompanied by temperature decreases in the lower stratosphere, and there is already some evidence of this effect in the polar regions. Temperature decreases in the lower stratosphere are very likely to increase the frequency and extent of polar stratospheric clouds (PSCs) that catalyze CFC–ozone reactions, and result in a strengthening of the polar vortex, which in turn is likely to lead to longer-lasting conditions for ozone depletion[2]. The minimum winter temperatures in the arctic stratosphere are very close to the threshold for the formation of PSCs and the chlorine reactions that lead to ozone loss, and the Arctic remains vulnerable to large-scale ozone depletion[3]. It is also possible that temperature increases could lead to increased zonal flow at mid-latitudes causing the polar vortex to be more stable, again favoring ozone depletion and a delay in the eventual recovery of the ozone layer[4]. Furthermore, as greenhouse gas concentrations increase, the tropical tropopause is very likely to become warmer, resulting in the transport of more water vapor into the stratosphere, which in turn is likely to lead to the formation of PSCs at higher temperatures[5]. Therefore, the ozone in the arctic stratosphere would be at greater risk of depletion (see Section 5.6.2 (Ultraviolet radiation effects on freshwater ecosystems in the Arctic) for further discussion).
The underwater UV radiation environment changes dramatically with a decrease in snow- and ice-cover duration, especially if this occurs during periods of greatest UV radiation flux and ozone depletion. Analyses of the effects of melting arctic sea and lake ice show that this process results in order-of-magnitude increases in biological UV radiation exposure that greatly exceed those caused by moderate ozone depletion (Box 8.9)[6]. Lake and river ice are relatively transparent to UV radiation because of CDOM exclusion from the ice during freeze-up[7]. Small changes in snow cover and white ice, however, can radically influence the below-ice UV radiation levels in arctic waters[8].
Box 8.9. Implications of changing snow and ice cover for ultraviolet radiation exposure ![]() The UV radiation-attenuating effect of ice and snow over the plume of the Great Whale River, in Hudson Bay, Northern Québec, immediately offshore from the river mouth (April 1999).The ice was 1 m thick with white ice at its surface, and the snow was 2 cm thick.The percentage transmittance values are for the penetration of each wavelength into the water beneath before and after the removal of the snow. Inset shows the ratio of transmittance through the ice in each wavelength after (without snow) relative to before (with snow) the 2 cm of snow was cleared from the ice (adapted from Vincent and Belzile[9] The warming northern climate is prolonging open water conditions.The loss of UV radiation-attenuating snow and ice earlier in the season, when water temperatures are still low but UV irradiances are maximal, is likely to be especially stressful for aquatic biota. As shown below, white ice (ice with air inclusions) has a strong attenuating= effect on PAR (visible light) and an even greater effect on UV-A and UV-B radiation. This snowclearing experiment on Hudson Bay showed that only 2 cm of snow reduced the below-ice exposure to UV radiation and to PAR by about a factor of three, with slightly greater effects at the shorter wavelengths |
In arctic aquatic environments, variations in suspended particulates, and especially CDOM, affect transmission of UV radiation (Box 8.10). These variations can be more important than ozone depletion in determining the UV radiation exposure in the water column of freshwater systems.
Box 8.10. Colored dissolved organic matter: the natural sunscreen in arctic lakes and rivers Colored dissolved organic matter (CDOM) is composed of humic and fulvic materials (average to low molecular weight) that are derived from terrestrial soils, vegetation, and microbial activities, and is known to be an effective protective screen against UV radiation for freshwater biota (e.g., plankton[10], amphibians [11].These compounds absorb UV-A and UV-B radiation and short-wavelength visible light, and in high concentrations such as in arctic rivers they stain the water yellow or brown. Colored dissolved organic matter is now known to be the primary attenuator of underwater UV radiation in subarctic and high-arctic lakes[12]; Toolik Lake, Alaska[13]; arctic ponds[14]; and arctic coastal seas influenced by river inflows[15].The concentrations of CDOM in natural waters are influenced by pH (acidification can cause a severe decline[16]), catchment morphology, runoff, and the type and extent of terrestrial vegetation.The latter aspects are especially dependent on climate. ![]() Ultraviolet (<400 nm) and blue-light (400–500 nm) radiation absorption by CDOM in the Mackenzie River (Inuvik, October 2002).The lower curves are for surface samples near the same date in the Beaufort Sea showing the CDOM influence at 2, 170, and 220 km offshore from the mouth of the river[17]. The paleoecological record has been helpful in examining past impacts of climate on biological underwater UV radiation exposure, specifically by using fossil diatoms in lake sediments as quantitative indicators of variations in CDOM.This record also underscores the |
In some areas of the Arctic, climate change is very likely to be accompanied by increased vegetation, a concomitant increase in CDOM loading[21], and reduced exposure to underwater UV radiation. These positive effects are likely, however, to be offset by reduced availability of PAR[22]. Marked south–north gradients in present-day CDOM concentrations in arctic waters are associated with the latitudinal distribution of terrestrial vegetation. Colored dissolved organic matter loading of freshwater systems is less pronounced at higher latitudes. Lakes in the tundra and polar-desert biomes contain low amounts of these materials; small variations in CDOM concentration in these systems can cause major changes in underwater UV radiation exposure[23]. Freshwaters in northern Scandinavia are low in dissolved carbon similar to water bodies in North America; the median DOC concentration for 25 lakes above the treeline in Finnish Lapland was 18 mg C/l[24]. Although acid precipitation enhances underwater UV radiation levels by reducing DOC concentrations in the water, increased thawing of permafrost with climate change is very likely to increase soil runoff and levels of DOC (or CDOM) in arctic freshwater systems. This is very likely to be accompanied by an increase in water turbidity, which will probably not only decrease PAR penetration but also increase the relative proportion of UV radiation, thereby hindering repair processes in aquatic organisms that are stimulated by longer wavelengths. Increased physical turbulence is also likely to expose planktonic organisms to unfavorable irradiance conditions (e.g., exposure to high levels of surface UV radiation and PAR), the effects of which are likely to be especially severe for species that cannot migrate.
Ultraviolet radiation effects on aquatic biota and ecosystems (8.6.2)
The effects of UV radiation in the aquatic environment range from molecular to whole-ecosystem. Photobiological damage includes the direct effects of UV radiation in which photons are absorbed by biological molecules such as nucleic acids and proteins that then undergo photochemical alteration. An alternative damage pathway is via the interaction of UV radiation and organic compounds or other photosensitizing agents to produce reactive oxygen species such as superoxide and hydroxyl radicals. These can diffuse away from the site of production and cause oxidative damage to enzymes, lipid membranes, and other cellular constituents.
Aquatic biota have four main lines of defense against UV radiation damage: escape, screening, quenching (chemical inactivation), and repair. The net stress imposed by the UV radiation environment reflects the energetic costs of protection and repair in addition to the rate of photochemical degradation or alteration of cellular components[25]. These defenses are well illustrated by arctic zooplankton, but despite this protection these organisms remain vulnerable to ambient UV radiation levels[26], particularly in the cold, shallow, CDOM-poor waters that characterize many arctic lakes and ponds.
Changes in underwater UV radiation exposure are likely to directly affect the species composition of aquatic biota at each trophic level, as well as cause effects that cascade throughout the benthic[27] or pelagic[28] food webs and the coupling between them. Some trophic responses are likely to be "bottom:up effects" in that UV radiation exposure reduces the quantity or quality of prey and thereby reduces food supply to the next level of consumer organisms. This could occur, for example, via shifts towards inedible or less digestible algal species[29] or by reducing the nutritional value of food organisms[30]. The effects of variations in UV-B radiation on the quality of phytoplankton photosynthetic products have received little attention except for studies at the pigment level[31]. Any alteration in the biochemical composition of primary producers is likely to change the nutritional value of food consumed by grazers (thus influencing energy flow throughout the food web) as well as restrict the production of photoprotective compounds against UV radiation. Short-term exposure to enhanced UV-B radiation levels in phytoplankton populations of various lakes in the Canadian High Arctic influenced the allocation of newly fixed carbon into the major macromolecular classes[32]. Generally, synthesis of both protein and polysaccharides was inhibited by enhanced UV-B radiation levels, and the photosynthate would remain or accumulate in the pool of low molecular weight compounds. Lipid synthesis was insensitive to UV-B radiation levels and represented the most conservative and uniform class, accounting for about 20% of total carbon fixed. Overall, these results were similar to those observed for Lake Ontario[33]. However, the various classes of lipid may respond differently to variations in UV-B radiation levels. For example, exposure to UV radiation influenced fatty acid composition in algal cultures[34]. Other studies observed that the effect of UV-B radiation on the major lipid classes is species-specific[35].
Higher trophic levels are dependent on phytoplankton either directly as food or indirectly via trophic cascades. Inhibition of growth and cell division in phytoplankton will most often affect the food quality of these cells by placing stoichiometric constraints on the grazer[36]. Hessen and Alstad Rukke[37] also showed that water hardness could be a major determinant of susceptibility to UV radiation damage among calcium-demanding species such as Daphnia.
They suggested that calcium, which is an important element for invertebrates with calcified exoskeletons, in low concentrations (low-pH lakes, acidification) could reduce the stress tolerance of organisms. Although several studies of freshwater invertebrate species have reported increased mortality in response to increased UV radiation levels, especially zooplankton[38], the variation in UV radiation tolerance is high among species and life stages[39]. In general, small zooplankton (small rotifers) are considered to have a high UV radiation tolerance, while large species vary in their tolerance both among and within species. Leech and Williamson[40] found that cladocerans had the lowest UV radiation tolerance and exhibited high variability among species. Daphnia was one of the most sensitive groups of organisms, while adult calanoid and cyclopoid copepods had high UV radiation tolerances. In a comparison of lakes across a successional gradient of catchment vegetation and thus CDOM content, three zooplankton species (Asplanchna priodonta, Ceriodaphnia quadrangula, and Bosmina longirostris) were absent from low-CDOM, UV-transparent waters, and perished when transplanted from a CDOM-rich lake in the series and held at 0.5 m depth under full UV radiation exposure in a clear lake. In contrast, two species that avoided high UV radiation exposure in the near-surface waters (Daphnia pulicaria and Cyclops scutifer, a highly UV-tolerant species) occurred in even the clearest lakes[41]. Morphotypic and biochemical differences among populations of a given species may also play an important role. Pigmented clones of Daphnia were more tolerant of UV radiation than transparent clones[42], and pigmentation appears to increase in response to increased UV radiation exposure (Box 8.11)[43]. Studies of the effects of natural and enhanced UV radiation levels on fish are rare, but laboratory experiments have shown that high-latitude species of trout have sunburns, increased fungal infections, and higher mortality when exposed to increased dosages of UV radiation[44].
Box 8.11. Ultraviolet radiation protection and recovery mechanisms in arctic freshwaters Aquatic organisms have varying abilities to counter the effects of UV radiation. Photoprotective and repair processes are particularly important in preventing and reversing UV radiation damage to photosynthetic mechanisms. A range of potential repair processes is stimulated by longer wavelengths to counteract the damaging effects of UV radiation.The relative importance of repair versus protection will vary depending on specific conditions and the physiological characteristics of the species assemblage[45]. Organisms living in arctic lakes have evolved several strategies to cope with UV radiation, which play an important role in shallow and highly UV-transparent arctic lakes and ponds. Some species of algae and zooplankton have an ability to reduce their exposure to UV radiation by vertical migration, which may be a response to high intensities of UV radiation[46]. Leech and Williamson[47] and Rhode et al.[48] provided further evidence that organisms avoid highly irradiated areas by escaping the brightly lit surface zone. ![]() Changes over time in solar radiation (solid line) and the rise in the UV-screening pigment melanin (data points) in the zooplankton Daphnia umbra in Lake Saanajärvi, northern Finland, immediately after ice-out[49]. In addition to avoidance, aquatic organisms can escape from UV radiation by reducing the effective radiation that penetrates the cell. A number of UV-protecting compounds have been described; the three major types are carotenoids, mycosporine-like amino acids (MAAs), and melanin.The photoprotective properties of carotenoids are mainly associated with antioxidant mechanisms and inhibition of free radicals, as opposed to direct UV radiation screening[50]. Carotenoids absorb wavelengths in the visible light spectrum and do not therefore provide direct protection from UV radiation. Mycosporine-like amino acids have absorption maxima ranging from 310 to 360 nm within the UV wavelength range.They are present in alpine phytoplankton and zooplankton[50] (Sommaruga and Garcia-Pichel, 1999;Tartarotti et al., 2001) and also occur in arctic freshwater organisms although there is no research on this. Animals are unable to synthesize MAAs and carotenoids, and must therefore acquire these compounds from their diet. Cladocera and fish produce melanin, with absorption maxima between 250 and 350 nm. Melanin acts by absorbing radiation before it enters the body tissues, and its synthesis seems to be a direct response to UV radiation[51]. Melanic zooplankton are typically found in clear arctic waters where the absorbance of UV radiation is low, and in shallow ponds where high DOC levels may not provide enough protection from UV radiation[52]. It has also been shown that melanin synthesis followed the annual variation in UV radiation levels (i.e., synthesis peaked at the time of maximum underwater UV irradiance, see figure). Aquatic organisms can also repair damage from UV radiation by nucleotide excision repair or by photoreactivation mechanisms, such as photoenzymatic repair[53]. Brief exposure to UV radiation triggers only the initial UV radiation stress response. However, responses over long periods show that organisms can acclimate to the UV radiation stress and/or recover growth rates with the development of photoprotective strategies (e.g. the synthesis of photoprotective compounds). A long-term enclosure experiment conducted in a high-arctic lake on Ellesmere Island (Nunavut, Canada) showed an initial decrease in phytoplankton productivity with enhanced UV-B radiation exposure, with recovery after 19 days[54]. Long-term acclimation to and recovery from increased levels of UV radiation were also observed in a cultured marine diatom[55]. Antecedent light conditions, temperature, nutrient availability, and/or variations between species are all factors that can affect acclimation of organisms to high intensities of UV-B radiation[56]. |
Other trophic responses are likely to be top-down effects, in which some species are released from grazing pressure or predation by UV inhibition of the consumers and thereby achieve higher population densities[57]. This complex combination of direct and indirect effects makes any future shifts in aquatic ecosystem structure extremely difficult to project. In addition, the deleterious effects of UV-B radiation at the community level are difficult to assess since they are generally species-specific. For example, Wickham and Carstens[58] showed that the responses of the planktonic microbial communities in Greenland ponds to ambient UV-B radiation levels varied greatly between species, especially rotifers and ciliates.
Multiple factors seem to affect amphibians negatively. These factors include both site-specific, local effects (e.g., pesticide deposition, habitat destruction, and disease) as well as global effects (e.g., increased UV-B radiation exposure and climate change[59]). Amphibians have been the focus of special interest at temperate latitudes because of the recent widespread decline in many frog populations and the recognized value of these organisms as sensitive indicators of environmental change. Although many amphibians can be relatively resistant to UV-B radiation, it can cause deformities, delays in development, behavioral responses, physiological stress, and death in frogs. The rise in UV-B radiation levels associated with stratospheric ozone depletion has been widely promoted as one of several hypotheses to account for their decline[60]. However, the effects are controversial and in many habitats where the frogs are declining, the animals are well protected by CDOM. A small number of frog species occur in the subarctic and the Arctic, including the common frog (Rana temporaria) and the wood frog (Rana sylvatica – North America), with distributions extending north of the Arctic Circle. Contrary to expectation, however, these populations may experience higher UV radiation exposures under natural conditions relative to temperate regions, and therefore be more pre-adapted, because of lower concentrations of UV-screening CDOM in high-latitude waters[61] and life-cycle characteristics (phenology: higher UV-B radiation doses during the breeding season at higher latitudes[62]). There is considerable variation in UV radiation tolerance between amphibian strains and species; for example, a latitudinal comparison in northern Sweden found that R. temporaria embryos were relatively tolerant of UV-B radiation, with no clear latitudinal differences[63]. The positive and negative effects of climate change on arctic habitats (e.g., duration of open water, extent of wetlands) are likely to have much greater impacts on amphibians than changes in UV radiation exposure.
Impacts on physical and chemical attributes (8.6.3)
The large arctic [[river]s] are relatively protected from UV radiation exposure because of their high CDOM content[64]. Conversely, natural and increased UV radiation levels are likely to be important for photochemical loss of carbon from these systems. For example, there is evidence that the duration of ice-free conditions has increased in the Mackenzie River, Canada[65] and the River Tornio, Finland. The resultant increased exposure to UV radiation is likely to favor increased annual rates of UV degradation of riverine DOC, with possible impacts on the inshore coastal waters that receive these inputs.
In addition to having low CDOM concentrations and resultant deep penetration of UV radiation, many arctic lakes, ponds, and wetlands are shallow systems. The mean measured depth for more than 900 lakes in northern Finland was less than 5 m[66], and was about 5 m for 31 lakes in the vicinity of Tuktoyaktuk (Northwest Territories)[67] and 46 lakes on Ellesmere Island (Nunavut)[68]. Consequently, all functional groups, including the benthos, are often exposed to UV radiation throughout the entire water column. In addition, many aquatic species stay in the offshore pelagic zone. Even species that are more benthic or littoral are protected minimally by macrophytes, as arctic waters, especially those in barren catchments, often contain little aquatic vegetation.
Changes in UV radiation exposure in these northern ecosystems are amplified by the low CDOM concentrations. Most have concentrations of DOC below 4 mg DOC/l, the threshold below which there are marked changes in UV radiation penetration through the water column, and in the ratio of wavelengths controlling the damage–repair balance, with only minor changes in CDOM[69].
The initial impacts of climate change are likely to be associated with the loss of permanent ice covers in the far northern lakes: this appears to have already recently taken place in the Canadian High Arctic[70]. These effects are likely to be amplified by prolonged open-water conditions in lakes and ponds. However, other physical changes in these environments (e.g., wind-induced mixing) are likely to have greater perturbation effects than those associated with increased UV radiation exposure. Although increases in CDOM are very likely to mitigate the effects of increased UV radiation levels, decreases in PAR are very likely to hamper photosynthesis. Furthermore, increased turbidity associated with thawing permafrost is likely to further reduce the exposure of organisms to damaging UV radiation[71].
The photochemical effects of increased UV radiation levels are also likely to influence the toxicity of contaminants (see also section 8.7). Mercury (Hg) is the principal toxic chemical of concern in the Arctic and elsewhere. Methyl mercury (MeHg) is the most toxic form and the only form that biomagnifies in food chains. It was recently shown that:
- ultraviolet radiation exposure photoreduces divalent mercury (Hg2+, the soluble form in lakes) to elemental mercury (Hg0, the form that can volatilize from lakes[72]);
- ultraviolet radiation can also influence photo-oxidation (the conversion of Hg0 to Hg2+[73]);
- the formation of MeHg in arctic wetlands is very sensitive to temperature;
- ultraviolet radiation photodegrades MeHg; and
- most of the Hg in recently fallen snow moves back to the atmosphere within a few days of exposure to solar radiation[74].
Not only is photochemical reduction of Hg important in the Arctic, but microbial reduction and oxidation may also occur as shown previously in temperate lake waters. Microbial oxidation is turned on by a hydrogen peroxide-dependent enzyme likely triggered by photochemical production of hydrogen peroxide[75]. The interactions between UV radiation, temperature, and pH can alter Hg mobilization and speciation, and regulate the levels of Hg in organisms at the base of the food web. Photochemical events during spring are likely to be especially sensitive to rising UV-B radiation levels. Large quantities of Hg are photochemically oxidized and precipitate out of the arctic atmosphere with the first sunlight each spring, resulting in a rapid rise in Hg concentrations in snow; 24-hour variations in these atmospheric Hg-depletion events correlate with fluctuations in UV-B irradiance[76]. Increasing spring levels of UV radiation due to stratospheric ozone depletion would probably enhance this so-called "mercury sunrise" phenomenon.
Wetlands and peatlands are rich in CDOM and the aquatic biota are therefore well protected from UV radiation exposure. Early loss of snow and ice, however, is likely to increase exposure during a critical growth phase. Photochemical processes may be especially active in these shallow waters, and this mechanism of CDOM loss is very likely to accelerate with temperature increases (snow-cover loss) and ozone depletion.
Impacts on biotic attributes (8.6.4)
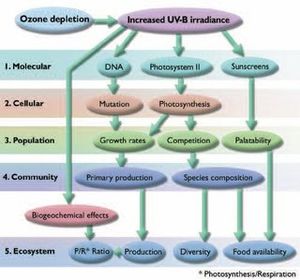
Mild increases in UV radiation levels are likely to stimulate biological processes via photochemical release of low molecular weight organic carbon substrates and nutrients. More severe increases are likely to cause damage and/or a shift toward UV-tolerant species with a potential loss of diversity or other unique ecosystem attributes (Fig. 8.20).
Rivers and streams (8.6.4.1)
Benthic mats and films are a common feature of high-latitude streams as well as many ponds, lakes, and wetlands, and are often dominated by cyanobacteria, especially the nitrogen-fixing genus Nostoc and filamentous species of the order Oscillatoriales[78]. These communities commonly occur in shallow water systems where UV radiation exposure is likely to be high. Ultraviolet radiation has a broad range of deleterious effects on benthic cyanobacteria including on their pigment content, nitrogenase activity, photosynthesis, and respiration[79]. Much of the literature, however, reports experiments conducted under unrealistically high UV radiation dosages provided by artificial lamps, and many of the effects are likely to be much less apparent or absent in natural ecosystems, even under conditions of severe ozone depletion[80]. Periphyton and benthic invertebrates are well protected given their avoidance and sunscreen capacities. Zooplankton and phytoplankton communities are well developed in large arctic rivers[81], however, they are generally protected by high CDOM concentrations in these waters.
Lakes, ponds, and wetlands (8.6.4.2)
In the Arctic, lake organisms have to cope with low nutrient conditions and/or low food availability, low temperatures, and short growing seasons (3–5 months). The UV radiation damage–repair balance may be especially sensitive to features of the arctic freshwater environment. Most of these ecosystems are oligotrophic and phytoplankton are therefore commonly limited by nutrient supply[82] in addition to low temperatures[83]. As a result, the photosynthetic rates per unit biomass tend to be extremely low, even in comparison with other low-temperature systems such as sea ice, polar oceans, and low-temperature cultures[84]. Because of the low temperatures and low nutrients, phytoplankton photosynthetic rates are extremely low in Canadian high-arctic lakes (usually less than 1.5 ?g C/?g Chl-a/hr) and phytoplankton perform very poorly under high-light regimes[85]. There have been few explicit tests of the effect of temperature on UV radiation damage of planktonic systems[86]. However, since enzymatic processes are temperature-dependent (whereas damage induction is not), the slow metabolic rates of northern phytoplankton are likely to have a direct effect on the net stress imposed by increased UV radiation exposure by reducing all cellular processes including the rate of repair of photochemical damage. In the antarctic waters, for example, low temperatures drastically reduce repair to the extent that algal cells failed to show any photosynthetic recovery for at least five hours after UV radiation exposure[87]. The low nutrient conditions that characterize northern lakes are likely to further compound this effect by reducing the availability of elemental resources for building enzyme systems involved in the functioning of the cell, including the repair of UV radiation damage, and are also likely to limit the investment in photoprotective mechanisms. Moreover, the lower temperatures probably reduce the affinity of the phytoplankton cells for nutrient uptake by membrane transport processes[88], thereby increasing nutrient limitation.
The paleoclimatic record has also provided insights into the possible effects of past climate change on UV radiation exposure in aquatic ecosystems. For instance, analyses of fossil diatom assemblages in northern and alpine lake sediments have indicated that variations in underwater UV irradiance during the Holocene had major impacts on algal community structure and productivity[89]. Paleo-optical studies from subarctic lakes have revealed large fluctuations in biologically damaging underwater UV irradiance over the last 6,000 years, accompanied by pronounced shifts in algal species composition and changes in the balance between benthic and pelagic primary producers[90]. Vinebrooke and Leavitt[91] observed similar effects in low-CDOM mountain lakes.
There are only a few studies of temperature-dependent UV radiation damage to zooplankton[92]. In general, it is assumed that low temperatures would slow down the UV radiation damage repair mechanisms such as DNA repair and detoxification of reactive oxygen species. However, contrary to expectations, Borgeraas and Hessen[93] found that reduced temperatures increased survival among UV-irradiated Daphnia. They argued that although repair mechanisms are slower in the cold, UV-triggered activation processes (such as reactive oxygen species metabolism and lipid peroxidation) also slow down with decreasing temperature, thereby increasing Daphnia survival.
At ambient levels, UV-B radiation can contribute up to 43% of the photoinhibition of photosystem II function in phytoplankton populations of Canadian high-arctic lakes (as measured by both in vivo and dichlorophenyldimethyl urea-enhanced fluorescence) as well as decreasing (by up to 40%) phytoplankton productivity rates near the water surface[94]. The smallest size fraction (i.e., picoplankton: 0.2–2 ?m) usually represents more than 50% of total phytoplankton productivity in high-arctic lakes[95]. Oligotrophic conditions tend to select for small cells with a high surface-to-volume ratio that favors nutrient transport at low substrate concentrations. Small cells are especially sensitive to UV radiation because they have high illuminated surface-to-volume ratios, little self-shading, and low effectiveness of screening pigments[96]. Even the production of UV radiation sunscreens is unlikely to confer much protection given the short path length in these cells[97], and studies of a variety of organisms have shown that enhanced UV radiation exposure inhibits the growth of larger cells less than that of smaller cells[98]. Laurion and Vincent[99] evaluated the size dependence of UV radiation effects on photosynthesis in subarctic lakes with a series of short-term photosynthetic experiments, which showed that, in contrast to expectations, the smaller cells were more resistant to UV radiation than larger cells. This smaller-cell fraction was dominated by cyanobacteria, a group known to have a broad range of effective UV-protective mechanisms[100]. Kaczmarska et al.[101] also found low UV radiation susceptibility in a picocyanobacteria-dominated phytoplankton assemblage from a clear lake in southern Canada. On the other hand, short-term experiments in several high-arctic lakes showed that the relative contribution of picoplankton (0.2–2 ?m) to phytoplankton production generally decreased with increased UV-B radiation exposure while the larger cells (>20 ?m) were more UV-B radiation tolerant and their contribution to productivity usually increased after UV-B radiation exposure. Arctic lake experiments at Spitsbergen also indicated a greater sensitivity of the picocyanobacteria relative to larger colonial species to UV-B radiation exposure[102]. A study by Boelen et al.[103] showed that for marine tropical plankton, UV-B-induced DNA damage was not significantly different between two size classes (0.2–0.8 ?m and 0.8–10 ?m). Given the variability in results between studies, other aspects such as species-specific sensitivity, repair capacities, or cell morphology might be more important than cell size[104].
The level of photoinhibition by UV radiation in phytoplankton can be modified by many factors that influence the extent of exposure within the water column. For instance, vertical mixing can affect the time and duration of phytoplankton exposure to UV radiation and diminish or aggravate projected inhibition of photosynthesis obtained under simulated conditions (continuous UV radiation exposures). In a well-mixed water column, the planktonic community can seek refuge from UV-B radiation, and photo-repair in the deeper portion of the column. However, the formation of near-surface thermoclines caused by high solar irradiance, calm [[wind]s], and solar heating of the surface water can retain the phytoplankton under high irradiances for longer periods of times[105] and result in UV radiation damages that can exceed what can be repaired[106]. During the spring and summer in the Arctic, climatic conditions (e.g., clouds, rain, snow, fog, and wind) that change tremendously from day-to-day can affect the amount of UV radiation exposure as well as the ratios of UV radiation to longer wavelengths reaching the surface of the earth. In the coastal areas of northern Norway, variation in cloudiness was demonstrated to influence UV radiation levels. The relative amount of UV-A and UV-B radiation to PAR increased during periods of heavy cloud cover[107] because clouds reflect and return radiation[108].
Ultraviolet radiation may impair the transfer of carbon from the microbial food web to higher trophic levels, including zooplankton and fish. However, increased photochemical activity associated with UV radiation also has the potential to stimulate some heterotrophic species by causing the breakdown of high molecular weight organic compounds into a more available form[109] that can then be taken up for bacterial and protist growth. A study using large enclosures in a high-arctic lake with high levels of dissolved organic compounds showed that after long-term exposure to enhanced UV-B radiation, heterotrophic bacterial production and zooplankton density increased, which may have resulted from an increase in nutrient availability caused by photodegradation of organic compounds and the stimulation of heterotrophic pathways[110].
Some wetland biota such as amphibians are known to be highly sensitive to UV radiation, although sensitivity varies greatly among populations, and other factors such as climate effects on habitat extent are likely to have much greater impacts on northern species (See Ultraviolet radiation effects on aquatic biota and ecosystems, above). A variety of complex responses have been observed to date in wetland plants. For example, UV-B radiation effects on the growth of high-latitude mosses appear to be a function of water supply as well as species. Field irradiation (UV-B radiation levels increased 15%) of Sphagnum fuscum caused a 20% reduction in growth, however, growth of the other moss species increased by up to 31% with the enhanced UV-B radiation. This stimulatory effect, however, ceased or was reversed under conditions of reduced water supply[111].
Chapter 8: Freshwater Ecosystems and Fisheries
8.1. Introduction (Ultraviolet radiation effects on freshwater ecosystems in the Arctic)
8.2. Freshwater ecosystems in the Arctic
8.3. Historical changes in freshwater ecosystems
8.4. Climate change effects
8.4.1. Broad-scale effects on freshwater systems
8.4.2. Effects on hydro-ecology of contributing basins
8.4.3. Effects on general hydro-ecology
8.4.4. Changes in aquatic biota and ecosystem structure and function
8.5. Climate change effects on arctic fish, fisheries, and aquatic wildlife
8.5.1. Information required to project responses of arctic fish
8.5.2. Approaches to projecting climate change effects on arctic fish populations
8.5.3. Climate change effects on arctic freshwater fish populations
8.5.4. Effects of climate change on arctic anadromous fish
8.5.5. Impacts on arctic freshwater and anadromous fisheries
8.5.6. Impacts on aquatic birds and mammals
8.6. Ultraviolet radiation effects on freshwater ecosystems
8.7. Global change and contaminants
8.8. Key findings, science gaps, and recommendations
References
Citation
Committee, I. (2012). Ultraviolet radiation effects on freshwater ecosystems in the Arctic. Retrieved from http://editors.eol.org/eoearth/wiki/Ultraviolet_radiation_effects_on_freshwater_ecosystems_in_the_Arctic- ↑ Vincent, W.F. and C. Belzile, 2003. Biological UV exposure in the polar oceans: Arctic-Antarctic comparisons. In: A.H.L. Huiskes, W.W.C. Gieskes, J. Rozema, R.M.L. Schorno, S.M. van der Vies and W.J. Wolff (eds.). Antarctic Biology in a Global Context. Proceedings of the VIIIth SCAR International Biology Symposium, 27 August –1 September 2001, pp. 176–181.
- ↑ Staehelin, J., N.R.P. Harris, C. Appenzeller and J. Eberhard, 2001. Ozone trends: a review. Reviews of Geophysics, 39:231–290.
- ↑ Dahlback, A., 2002. Recent changes in surface UV radiation and stratospheric ozone at a high arctic site. In: D.O. Hessen (ed.). UV Radiation and Arctic Ecosystems. Ecological Studies, 153:3–22.
- ↑ Shindell, D.T., D. Rind and P. Lonergan, 1998. Increased polar stratospheric ozone losses and delayed eventual recovery owing to increasing greenhouse-gas concentrations. Nature, 392:589–592.
- ↑ Kirk-Davidoff, D.B., E.J. Hintsa, J.G. Anderson and D.W. Keith, 1999. The effect of climate change on ozone depletion through changes in stratospheric water vapour. Nature, 402:399–401.
- ↑ Vincent, W.F. and C. Belzile, 2003, Op. cit.
- ↑ Belzile, C., J.A.E. Gibson and W.F. Vincent, 2002a. Colored dissolved organic matter and dissolved organic carbon exclusion from lake ice: implications for irradiance transmission and carbon cycling. Limnology and Oceanography, 47:1283–1293.
- ↑ Belzile, C., W.F. Vincent, J.A.E. Gibson and P.Van Hove, 2001. Biooptical characteristics of the snow, ice and water column of a perennially ice-covered lake in the high Arctic. Canadian Journal of Fisheries and Aquatic Sciences, 58:2405–2418.
- ↑ Vincent and Belzile, 2003, Op. cit.
- ↑ Vincent,W.F. and S. Roy, 1993. Solar ultraviolet-B radiation and aquatic primary production: damage, protection, and recovery. Environmental Reviews, 1:1–12.
- ↑ Palen,W.J., D.E. Schindler, M.J. Adams, C.A. Pearl, R.B. Bury and S.A. Diamond, 2002. Optical characteristics of natural waters protect amphibians from UV-B in the U.S. Pacific Northwest. Ecology, 83:2951–2957.
- ↑ Laurion, I.,W.F.Vincent and D.R.S. Lean, 1997. Underwater ultraviolet radiation: development of spectral models for northern high latitude lakes. Photochemistry and Photobiology, 65:107–114.
- ↑ Morris, D.P., H.E. Zagarese, C.E.Williamson, E.G. Balseiro, B.R. Hargreaves, B. Modenutti, R. Moeller and C. Queimalinos, 1995. The attenuation of solar UV radiation in lakes and the role of dissolved organic carbon. Limnology and Oceanography, 40:1381–1391.
- ↑ Rautio, M. and A. Korhola, 2002a. Effects of ultraviolet radiation and dissolved organic carbon on the survival of subarctic zooplankton. Polar Biology, 25:460–468.
- ↑ Gibson, J.A.E.,W.F.Vincent, B. Nieke and R. Pienitz, 2000. Control of biological exposure to UV radiation in the Arctic Ocean: comparison of the roles of ozone and riverine dissolved organic matter. Arctic, 53:372–382;-- Vincent and Belzile, 2003, Op. cit.
- ↑ Schindler, D.W., P.J. Curtis, B.R. Parker and M.P. Stainton, 1996b. Consequences of climate warming and lake acidification for UV-B penetration in North American boreal lakes. Nature, 379:705–708.
- ↑ (W.F. Vincent and L. Retamal, Laval University, Québec City, unpubl. data, 2004)
- ↑ Ponader, K., R. Pienitz,W.F.Vincent and K. Gajewski, 2002. Limnological conditions in a subarctic lake (Northern Québec, Canada) during the late Holocene: analyses based on fossil diatoms. Journal of Paleolimnology, 27:353–366;-- Saulnier-Talbot, É., R. Pienitz and W.F.Vincent, 2003. Holocene lake succession and palaeo-optics of a subarctic lake, northern Québec, Canada.The Holocene, 13:517–526.
- ↑ Pienitz, R. and W.F.Vincent, 2000. Effect of climate change relative to ozone depletion on UV exposure in subarctic lakes. Nature,404:484–487.
- ↑ Saulnier-Talbot et al. (2003), Op. cit.
- ↑ Freeman, C., C.D. Evans, D.T. Monteith, B. Reynolds and N. Fenner, 2001. Export of organic carbon from peat soils. Nature, 412:785.
- ↑ Arrigo, K.R. and C.W. Brown, 1996. Impact of chromophoric dissolved organic matter on UV inhibition of primary productivity in the sea. Marine Ecology Progress Series, 140:207–216.–Neale, P.J., 2001. Modeling the effects of ultraviolet radiation on estuarine phytoplankton production: impact of variations in exposure and sensitivity to inhibition. Journal of Photochemistry and Photobiology, 62:1–8.–Pienitz, R. and W.F. Vincent, 2000. Effect of climate change relative to ozone depletion on UV exposure in subarctic lakes. Nature, 404:484–487.
- ↑ Laurion, I., W.F. Vincent and D.R.S. Lean, 1997. Underwater ultraviolet radiation: development of spectral models for northern high latitude lakes. Photochemistry and Photobiology, 65:107–114.–Vincent, W.F. and R. Pienitz, 1996. Sensitivity of high latitude freshwater ecosystems to global change: temperature and solar ultraviolet radiation. Geoscience Canada, 23:231–236.
- ↑ Rautio, M. and A. Korhola, 2002b. UV-induced pigmentation in subarctic Daphnia. Limnology and Oceanography, 47:295–299.
- ↑ Vincent, W.F. and P.J. Neale, 2000. Mechanisms of UV damage to aquatic organisms. In: S.J. de Mora, S. Demers and M. Vernet (eds.). The Effects of UV Radiation in the Marine Environment, pp. 149–176. Cambridge University Press.
- ↑ Zellmer, I.D., 1998. The effects of solar UVA and UVB on subarctic Daphnia pulicaria in its natural habitat. Hydrobiologia, 379:55–62.
- ↑ Bothwell, M.L., D.M.J. Sherbot and C.M. Pollock, 1994. Ecosystem response to solar ultraviolet-B radiation: influence of trophic-level interactions. Science, 265:97–100.
- ↑ Mostajir, B., S. Demers, S.J. de Mora, C. Belzile, J.-P. Chanut, M. Gosselin, S. Roy, P.Z.Villegas, J. Fauchot, J. Bouchard, D.F. Bird, P. Monfort and M. Levasseur, 1999. Experimental test of the effect of ultraviolet-B radiation in a planktonic community. Limnology and Oceanography, 44:586–596.
- ↑ Van Donk, E. and D.O. Hessen, 1995. Reduced digestibility of UV-B stressed and nutrient-limited algae by Daphnia magna. Hydrobiologia, 307:147–151.–Van Donk, E., B.A. Faafeng, H.J. De Lange and D.O. Hessen, 2001. Differential sensitivity to natural ultraviolet radiation among phytoplankton species in Arctic lakes (Spitsbergen, Norway). Plant Ecology, 154:247–259.
- ↑ Scott, J.D., L. Chalker-Scott, A.E. Foreman and M. D'Angelo, 1999. Daphnia pulex fed UVB-irradiated Chlamydomonas reinhardtii show decreased survival and fecundity. Photochemistry and Photobiology, 70:308–313.
- ↑ Buma, A.G.J., H.J. Zemmelink, K. Sjollema and W.W.C. Gieskes, 1996. UVB radiation modifies protein and photosynthetic pigment content, volume and ultrastructure of marine diatoms. Marine Ecology Progress Series, 142:47–54.–Zudaire, L. and S. Roy, 2001. Photoprotection and long-term acclimation to UV radiation in the marine diatom Thalassiosira weissflogi. Journal of Photochemistry and Photobiology B, 62:26–34.
- ↑ Perin, S.L., 2003. Influences of UVB radiation on lake ecosystems of High Arctic lakes. Ph.D Thesis, University of Ottawa.
- ↑ Smith, R.E.H., J.A. Furgal and D.R.S. Lean, 1998. The short-term effects of solar ultraviolet radiation on phytoplankton photosynthesis and photosynthate allocation under contrasting mixing regimes in Lake Ontario. Journal of Great Lakes Research, 24:427–441.
- ↑ Goes, J.I., N. Handa, S.Taguchi and T. Hama, 1994. Effect of UV-B radiation on the fatty acid composition of the marine phytoplankton Tretraselmis sp.: relation to cellular pigments. Marine Ecology Progress Series, 114:259–274.–Wang, K.S. and T.-J. Chai, 1994. Reduction in omega-3 fatty acids by UV-B radiation in microalgae. Journal of Applied Phycology, 6:415–421.
- ↑ De Lange, H.J. and E.Van Donk, 1997. Effects of UVB-irradiated algae on life history traits of Daphnia pulex. Freshwater Biology, 38:711–720.
- ↑ Hessen, D.O., H.J. De Lange and E. Van Donk, 1997. UV-induced changes in phytoplankton cells and its effects on grazers. Freshwater Biology, 38:513–524.
- ↑ Hessen, D.O. and N. Alstad Rukke, 2000. UV radiation and low calcium as mutual stressors for Daphnia. Limnology and Oceanography, 45:1834–1838.
- ↑ Hurtubise, R.D., J.E. Havel and E.E. Little, 1998.The effects of ultraviolet-B radiation on freshwater invertebrates: experiments with a solar simulator. Limnology and Oceanography, 43:1082–1088.–Leech, D.M. and C.E. Williamson, 2000. Is tolerance to UV radiation in zooplankton related to body size, taxon, or lake transparency? Ecological Applications, 10:1530–1540.–Rautio, M. and A. Korhola, 2002a. Effects of ultraviolet radiation and dissolved organic carbon on the survival of subarctic zooplankton. Polar Biology, 25:460–468.–Siebeck, O. and U. Böhm, 1994. Challenges for an appraisal of UV–B effects upon planktonic crustaceans under natural radiation conditions with a non-migrating (Daphnia pulex obtusa) and a migrating cladoceran (Daphnia galeata). In: C.E. Williamson and H.E. Zagarese (eds.). Impact of UV–B Radiation on Pelagic Freshwater Ecosystems. Archiv für Hydrobiologie - Advances in Limnology, 43:197–206.–Vinebrooke, R.D. and P.R. Leavitt, 1999a. Differential responses of littoral communities to ultraviolet radiation in an alpine lake. Ecology, 80:223–237.–Williamson, C.E., H. Zagarese, P.C. Schulze, B.R. Hargreaves and J. Seva, 1994.The impact of short-term exposure to UV-B radiation on zooplankton communities in north temperate lakes. Journal of Plankton Research, 16:205–218.
- ↑ Leech, D.M. and C.E. Williamson, 2000. Is tolerance to UV radiation in zooplankton related to body size, taxon, or lake transparency? Ecological Applications, 10:1530–1540.
- ↑ Leech, D.M. and C.E. Williamson, 2000. Is tolerance to UV radiation in zooplankton related to body size, taxon, or lake transparency? Ecological Applications, 10:1530–1540.
- ↑ Williamson, C.E., O.G. Olson, S.E. Lott, N.D. Walker, D.R. Engstrom and B.R. Hargreaves, 2001. Ultraviolet radiation and zooplankton community structure following deglaciation in Glacier Bay, Alaska. Ecology, 82:1748–1760.
- ↑ Hessen, D.O., J. Borgeraas, K. Kessler and U.H. Refseth, 1999. UV-B susceptibility and photoprotection of Arctic Daphnia morphotypes. Polar Research, 18:345–352.
- ↑ Rautio, M. and A. Korhola, 2002b. UV-induced pigmentation in subarctic Daphnia. Limnology and Oceanography, 47:295–299.
- ↑ Little, E.E. and D.L. Fabacher, 1994. Comparative sensitivity of rainbow trout and two threatened salmonids, Apache trout and Lahontan cutthroat trout, to ultraviolet-B radiation. In: C.E.Williamson and H.E. Zagarese (eds.). Impact of UV-B Radiation on Pelagic Freshwater Ecosystems. Archiv für Hydrobiologie - Advances in Limnology, 43:217–226.
- ↑ Banaszak, A.T. and P.J. Neale, 2001. Ultraviolet radiation sensitivity of photosynthesis in phytoplankton from an estuarine environment. Limnology and Oceanography, 46:592–603.
- ↑ Huntsman, A.G., 1924. Limiting factors for marine animals, II:The lethal effect of sunlight. Contributions to Canadian Biology, 2:83–88.
- ↑ Leech, D.M. and C.E.Williamson, 2001. In situ exposure to ultraviolet radiation alters the depth distribution of Daphnia. Limnology and Oceanography, 46:416–420.
- ↑ Rhode, S.C., M. Pawlowski and R.Tollrian, 2001.The impact of ultraviolet radiation on the vertical distribution of zooplankton of the genus Daphnia. Nature, 412:69–72.
- ↑ Adapted from Rautio and Korhola, 2002b, Op. cit.
- ↑ 50.0 50.1 Hessen, D.O., 1994. Daphnia responses to UV-light. In: C.E. Williamson and H.E. Zagarese (eds.). Impact of UV-B Radiation onPelagic Freshwater Ecosystems. Archiv für Hydrobiologie – Advances in Limnology, 43:185–195.
- ↑ Hobæk, A. and H.G.Wolf, 1991. Ecological genetics of Norwegian Daphnia. II. Distribution of Daphnia longispina genotypes in relation to short-wave radiation and water colour. Hydrobiologia,225:229–243.
- ↑ Hebert, P.D.N. and C.J. Emery, 1990.The adaptive significance of cuticular pigmentation in Daphnia. Functional Ecology, 4:703–710.;-- Rautio and Korhola, 2002b, Op. cit.
- ↑ Leech, D.M. and C.E.Williamson, 2000. Is tolerance to UV radiation in zooplankton related to body size, taxon, or lake transparency? Ecological Applications, 10:1530–1540.
- ↑ Perin, S.L., 2003. Influences of UVB radiation on lake ecosystems of High Arctic lakes. Ph.D Thesis, University of Ottawa.
- ↑ Zudaire, L. and S. Roy, 2001. Photoprotection and long-term acclimation to UV radiation in the marine diatom Thalassiosira weissflogi. Journal of Photochemistry and Photobiology B, 62:26–34.
- ↑ Ibid.
- ↑ Bothwell, M.L., D.M.J. Sherbot and C.M. Pollock, 1994. Ecosystem response to solar ultraviolet-B radiation: influence of trophic-level interactions. Science, 265:97–100.
- ↑ Wickham, S. and M. Carstens, 1998. Effects of ultraviolet-B radiation on two Arctic microbial food webs. Aquatic Microbial Ecology, 16:163–171.
- ↑ Häder, D.-P., H.D. Kumar, R.C. Smith and R.C. Worrest, 2003. Aquatic ecosystems: effects of solar ultraviolet radiation and interactions with other climatic change factors. In: J.F. Bornman, K. Solomon, and J.C. van der Leun (eds.). Environmental Effects of Ozone Depletion and its Interactions with Climate Change: 2002 Assessment. Photochemical and Photobiological Sciences, 2:39–50.
- ↑ Collins, J.P. and A. Storfer, 2003. Global amphibian declines: sorting the hypotheses. Diversity and Distributions, 9:89–98.
- ↑ Palen, W.J., D.E. Schindler, M.J. Adams, C.A. Pearl, R.B. Bury and S.A. Diamond, 2002. Optical characteristics of natural waters protect amphibians from UV-B in the U.S. Pacific Northwest. Ecology, 83:2951–2957.
- ↑ Merilä, J., M. Pahkala and U. Johanson, 2000. Increased ultraviolet-B radiation, climate change and latitudinal adaptation – a frog perspective. Annales Zoologici Fennici, 37:129–134.
- ↑ Pahkala, M., A. Laurila and J. Merilä, 2002. Effects of ultraviolet-B radiation on common frog Rana temporaria embryos from along a latitudinal gradient. Oecologia, 133:458–465.
- ↑ Gibson, J.A.E., W.F. Vincent, B. Nieke and R. Pienitz, 2000. Control of biological exposure to UV radiation in the Arctic Ocean: comparison of the roles of ozone and riverine dissolved organic matter. Arctic, 53:372–382.
- ↑ Magnuson, J.J., D.M. Robertson, B.J. Benson, R.H. Wynne, D.M. Livingstone, T. Arai, R.A. Assel, R.G. Barry, V. Card, E. Kuusisto, N.G. Granin, T.D. Prowse, K.M. Stewart and V.S. Vuglinski, 2000. Historical trends in lake and river ice cover in the Northern Hemisphere. Science, 289:1743–1746.
- ↑ Blom, T., A. Korhola and J. Weckström, 1998. Physical and chemical characterisation of small subarctic lakes in Finnish Lapland with special reference to climate change scenarios. In: R. Lemmelä and N. Helenius (eds.). Proceedings of the Second International Conference on Climate Change and Water, Espoo, Finland, 17–20 August 1998, pp. 576–587. Helsinki University.
- ↑ Pienitz, R. and J.P. Smol, 1994. The ecology and physicochemical characteristics of lakes in the subarctic and arctic regions of the Yukon Territory, Fennoscandia (Finland, Norway), the Northwest Territories and Northern Quebec. In: P.B. Hamilton (ed.). Proceedings of the Fourth Arctic-Antarctic Diatom Symposium, pp. 31–43. Canadian Technical Report of Fisheries and Aquatic Sciences No. 1957.
- ↑ Hamilton, P.B., D.R.S. Lean and M. Poulin, 1994.The physicochemical characteristics of lakes and ponds from the Northern regions of Ellesmere Island. In: P.B. Hamilton (ed.). Proceedings of the Fourth Arctic-Antarctic Diatom Symposium, pp. 57–63. Canadian Technical Report of Fisheries and Aquatic Sciences No. 1957.
- ↑ Laurion, I., W.F. Vincent and D.R.S. Lean, 1997. Underwater ultraviolet radiation: development of spectral models for northern high latitude lakes. Photochemistry and Photobiology, 65:107–114.
- ↑ Belzile, C., J.A.E. Gibson and W.F. Vincent, 2002a. Colored dissolved organic matter and dissolved organic carbon exclusion from lake ice: implications for irradiance transmission and carbon cycling. Limnology and Oceanography, 47:1283–1293.
- ↑ Belzile, C., W.F. Vincent and M. Kumagai, 2002b. Contribution of absorption and scattering to the attenuation of UV and photosynthetically available radiation in Lake Biwa. Limnology and Oceanography, 47:95–107.
- ↑ Amyot, M., D.R.S. Lean and G. Mierle, 1997. Photochemical formation of volatile mercury in high Arctic lakes. Environmental Toxicology and Chemistry, 16:2054–2063.
- ↑ Lalonde, J.D., M. Amyot, A.M.L. Kraepiel and F.M.M. Morel, 2001. Photooxidation of Hg(0) in artificial and natural waters. Environmental Science and Technology, 35:1367–1372.
- ↑ Lalonde, J.D., A.J. Poulain and M. Amyot, 2002.The role of redox reactions in snow on snow-to-air Hg transfer. Environmental Science and Technology, 36:174–178.
- ↑ Scully, N.M., W.F. Vincent, D.R.S. Lean and W.J. Cooper, 1997. Implications of ozone depletion for surface-water photochemistry: sensitivity of clear lakes. Aquatic Sciences, 59:260–274.
- ↑ Lindberg, S.E., S. Brooks, C.-J. Lin, K.J. Scott, M.S. Landis, R.K. Stevens, M. Goodsite and A. Richter, 2002. Dynamic oxidation of gaseous mercury in the arctic troposphere at polar sunrise. Environmental Science and Technology, 36:1245–1256.
- ↑ Vincent, W.F. and S. Roy, 1993. Solar ultraviolet-B radiation and aquatic primary production: damage, protection, and recovery. Environmental Reviews, 1:1–12.
- ↑ Vincent, W.F., 2000. Cyanobacterial dominance in the polar regions. In: B.A. Whitton and M. Potts (eds.).The Ecology of Cyanobacteria: Their Diversity in Time and Space, pp. 321–340. Kluwer Academic Publishers.
- ↑ Castenholz, R.W. and F. Garcia-Pichel, 2000. Cyanobacterial responses to UV-radiation. In: B.A. Whitton and M. Potts (eds.).The Ecology of Cyanobacteria: Their Diversity in Time and Space, pp. 591–614. Kluwer Academic Publishers.Vincent, W.F. and A. Quesada, 1994. Cyanobacterial responses to UV radiation: implications for Antarctic microbial ecosystems. In: C.S.–Weiler and P.A. Penhale (eds.). Ultraviolet Radiation in Antarctica: Measurement and Biological Effects. American Geophysical Union, Antarctic Research Series, Vol. 62, pp. 111–124.
- ↑ Vincent, W.F. and P.J. Neale, 2000. Mechanisms of UV damage to aquatic organisms. In: S.J. de Mora, S. Demers and M. Vernet (eds.). The Effects of UV Radiation in the Marine Environment, pp. 149–176. Cambridge University Press.
- ↑ Rae, R. and W.F. Vincent, 1998a. Effects of temperature and ultraviolet radiation on microbial food web structure: potential responses to global change. Freshwater Biology, 40:747–758.
- ↑ Bergeron, M. and W.F. Vincent, 1997. Microbial food web responses to phosphorus supply and solar UV radiation in a subarctic lake. Aquatic Microbial Ecology, 12:239–249.
- ↑ Rae, R. and W.F. Vincent, 1998a. Effects of temperature and ultraviolet radiation on microbial food web structure: potential responses to global change. Freshwater Biology, 40:747–758.
- ↑ Markager, S., W.F. Vincent and E.P.Y. Tang, 1999. Carbon fixation by phytoplankton in high Arctic lakes: implications of low temperature for photosynthesis. Limnology and Oceanography, 44:597–607.
- ↑ Kalff, J. and H.E. Welch, 1974. Phytoplankton production in Char Lake, a natural polar lake, and in Meretta Lake, a polluted polar lake, Cornwallis Island, Northwest Territories. Journal of the Fisheries Research Board of Canada, 31:621–636.–Perin, S.L., 2003. Influences of UVB radiation on lake ecosystems of High Arctic lakes. Ph.D Thesis, University of Ottawa.–Rigler, F.H., 1978. Limnology in the high Arctic: a case study of Char Lake. Verheissungen der Internationale Vereinigung der gesamten Limnologie, 20:127–140.
- ↑ Rae, R. and W.F. Vincent, 1998a. Effects of temperature and ultraviolet radiation on microbial food web structure: potential responses to global change. Freshwater Biology, 40:747–758.
- ↑ Neale, P.J., R.F. Davis and J.J. Cullen, 1998. Interactive effects of ozone depletion and vertical mixing on photosynthesis of Antarctic phytoplankton. Nature, 392:585–589.
- ↑ Nedwell, D.B., 2000. Life in the cooler – starvation in the midst of plenty; and implications for microbial polar life. In: C.R. Bell, M. Brylinski and P. Johnson-Green (eds.). Proceedings of the 8th International Symposium on Microbial Ecology, pp. 299–305. Atlantic Canada Society for Microbiology, Halifax.
- ↑ Leavitt, P.R., R.D. Vinebrooke, D.B. Donald, J.P. Smol and D.W. Schindler, 1997. Past ultraviolet radiation environments in lakes derived from fossil pigments. Nature, 388:457–459.–Pienitz, R. and W.F. Vincent, 2000. Effect of climate change relative to ozone depletion on UV exposure in subarctic lakes. Nature, 404:484–487.
- ↑ Pienitz, R. and W.F. Vincent, 2000. Effect of climate change relative to ozone depletion on UV exposure in subarctic lakes. Nature, 404:484–487.
- ↑ Vinebrooke, R.D. and P.R. Leavitt, 1999b. Phytobenthos and phytoplankton as potential indicators of climate change in mountain lakes and ponds: a HPLC-based pigment approach. Journal of the North American Benthological Society, 18:14–33.
- ↑ Borgeraas, J. and D.O. Hessen, 2000. UV-B induced mortality and antioxidant enzyme activities in Daphnia magna at different oxygen concentrations and temperatures. Journal of Plankton Research, 22:1167–1183.
- ↑ Borgeraas, J. and D.O. Hessen, 2000. UV-B induced mortality and antioxidant enzyme activities in Daphnia magna at different oxygen concentrations and temperatures. Journal of Plankton Research, 22:1167–1183.
- ↑ Perin, S.L., 1994. Short-term influences of ambient UV-B radiation on phytoplankton productivity and chlorophyll fluorescence in two lakes of the High Arctic. M.Sc. Thesis, Trent University, Ontario.
- ↑ Perin, S.L., 1994. Short-term influences of ambient UV-B radiation on phytoplankton productivity and chlorophyll fluorescence in two lakes of the High Arctic. M.Sc. Thesis, Trent University, Ontario.
- ↑ Karentz, D., J.E. Cleaver and D.L. Mitchell, 1991. Cell survival characteristics and molecular responses of Antarctic phytoplankton to ultraviolet-B radiation. Journal of Phycology, 27:326–341.–Raven, J.A., 1998.The twelfth Tansley Lecture. Small is beautiful: the picophytoplankton. Functional Ecology, 12:503–513.
- ↑ Garcia-Pichel, F., 1994. A model for internal self-shading in planktonic organisms and its implications for the usefulness of ultraviolet sunscreens. Limnology and Oceanography, 39:1704–1717.
- ↑ Karentz, D., J.E. Cleaver and D.L. Mitchell, 1991. Cell survival characteristics and molecular responses of Antarctic phytoplankton to ultraviolet-B radiation. Journal of Phycology, 27:326–341.
- ↑ Laurion, I. and W.F. Vincent, 1998. Cell size versus taxonomic composition as determinants of UV sensitivity in natural phytoplankton communities. Limnology and Oceanography, 43:1774–1779.
- ↑ Vincent, W.F., 2000. Cyanobacterial dominance in the polar regions. In: B.A.Whitton and M. Potts (eds.).The Ecology of Cyanobacteria: Their Diversity in Time and Space, pp. 321–340. Kluwer Academic Publishers.
- ↑ Kaczmarska, I., T.A. Clair, J.M. Ehrman, S.L. MacDonald, D.R.S. Lean and K.E. Day, 2000.The effect of ultraviolet B on phytoplankton populations in clear and brown temperate Canadian lakes. Limnology and Oceanography, 45:651–663.
- ↑ Van Donk, E., B.A. Faafeng, H.J. De Lange and D.O. Hessen, 2001. Differential sensitivity to natural ultraviolet radiation among phytoplankton species in Arctic lakes (Spitsbergen, Norway). Plant Ecology, 154:247–259.
- ↑ Boelen, P., M.J.W. Veldhuis and A.G.J. Buma, 2001. Accumulation and removal of UVBR-induced DNA damage in marine tropical plankton subjected to mixed and simulated non-mixed conditions. Aquatic Microbial Ecology, 24:265–274.
- ↑ Boelen, P., M.J.W. Veldhuis and A.G.J. Buma, 2001. Accumulation and removal of UVBR-induced DNA damage in marine tropical plankton subjected to mixed and simulated non-mixed conditions. Aquatic Microbial Ecology, 24:265–274.
- ↑ Milot-Roy, V. and W.F. Vincent, 1994. UV radiation effects on photosynthesis: the importance of near-surface thermoclines in a subarctic lake. In: C.E.Williamson and H.E. Zagarese (eds.). Impact of UV-B Radiation on Pelagic Freshwater Ecosystems. Archiv für Hydrobiologie - Advances in Limnology, 43:171–184.
- ↑ Xenopoulos, M.A., Y.T. Prairie and D.F. Bird, 2000. Influence of ultraviolet-B radiation, stratospheric ozone variability, and thermal stratification on the phytoplankton biomass dynamics in a mesohumic lake. Canadian Journal of Fisheries and Aquatic Sciences, 57:600–609.
- ↑ Eilertsen, H.C. and O. Holm-Hansen, 2000. Effects of high latitude UV radiation on phytoplankton and nekton modeled from field measurements by simple algorithms. Polar Research, 19:173–182.
- ↑ Madronich, S., R.L. McKenzie, L.O. Björn and M.M. Caldwell, 1995. Changes in ultraviolet radiation reaching the Earth's surface. Ambio, 24:143–152.
- ↑ Bertilsson, S., R. Stepanauskas, R. Cuadros-Hansson, W. Granéli, J.Wikner and L. Tranvik, 1999. Photochemically induced changes in bioavailable carbon and nitrogen pools in a boreal watershed. Aquatic Microbial Ecology, 19:47–56.– Lindell, M.J., W. Granéli and L.J. Tranvik, 1996. Effects of sunlight on bacterial growth in lakes of different humic content. Aquatic Microbial Ecology, 11:135–141.– Reche, I., M.L. Pace and J.J. Cole, 1998. Interactions of photobleaching and inorganic nutrients in determining bacterial growth on colored dissolved organic carbon. Microbial Ecology, 36:270–280.– Wetzel, R.G., P.G. Hatcher and T.S. Bianchi, 1995. Natural photolysis by ultraviolet irradiance of recalcitrant dissolved organic matter to simple substrates for rapid bacterial metabolism. Limnology and Oceanography, 40:1369–1380.
- ↑ Perin, S.L., 2003. Influences of UVB radiation on lake ecosystems of High Arctic lakes. Ph.D Thesis, University of Ottawa.
- ↑ Gehrke, C., U. Johanson, D. Gwynn-Jones, L.O. Björn, T.V. Callaghan and J.A. Lee, 1996. Effects of enhanced ultraviolet-B radiation on terrestrial subarctic ecosystems and implications for interactions with increased atmospheric CO2. In: P.S. Karlsson and T.V. Callaghan (eds.). Plant Ecology in the Sub-Arctic Swedish Lapland. Ecological Bulletin, 45:192–203.